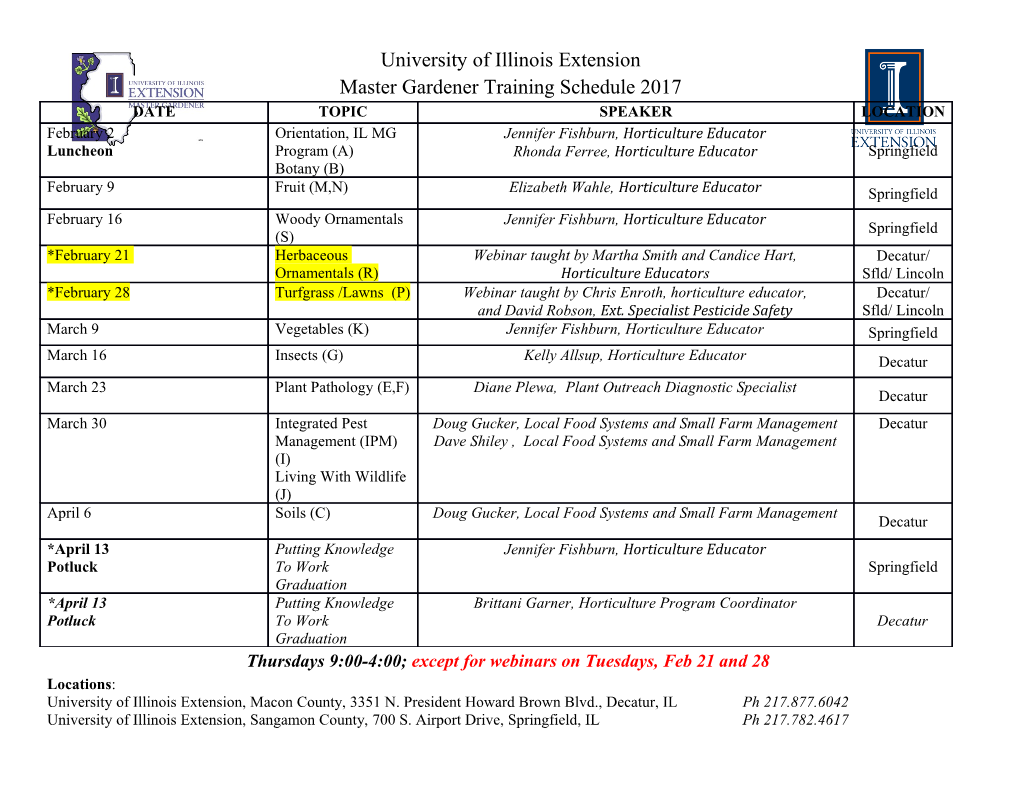
Case Study: Titin Ig domains Mu Gao and Eric Lee A Molecular Rubber Band In the extreme sport of bungee jumping, a daring athlete leaps from a great height and free- falls while a tethered cord tightens and stretches to absorb the energy from the descent. The bungee cord protects the jumper from serious injury, because its elasticity allows it to extend and provide a cushioning force that opposes gravity during the fall. Amazingly, nature also uses elasticity to dampen biological forces at the molecular level, such as during extension of a muscle fiber under stress. The molecular bungee cord that serves this purpose in the human muscle fiber is the protein titin, which functions to protect muscle fibers from damage due to overstretching. In this case study, we will examine how experimental and theoretical studies yield insight into the molecular mechanism for titin’s elasticity. 1 These are the files you will need to complete the exercises in this case study. 2 The Muscle Fiber and Titin Striated muscle, such as a skeletal or cardiac muscle, is a complex of elongated fibers arranged in parallel order and held together with connective tissue. These muscle fibers are typically 2 to 3 cm long and 100 µm in diameter. Each fiber consists of thread-like fibrils (1-2 µm diameter) known as myofibrils, which primarily contain thick filaments (12-18 nm diameter) and thin filaments (5-8 nm diameter). The thick filaments are structures built primarily from overlapping myosin proteins, which have globular heads that form cross bridges connected to thin filaments formed mainly by actin bundles. Viewed lengthwise, the myofibril is seen as a series of bands of alternating composition, separated by line-like regions (Fig 1a and b). The A band is a set of stacked thick filaments centered on the M line, overlapped on both sides by thin filaments, which compose the I band. In between each I band, a dense vertical region called the Z line divides the myofibrils into a functional unit called the sarcomere, which is the smallest self-contained contractile unit (resting length 2 to 3 µm) of the striated muscle. Figure 1: (a) The electron microscope image of striated muscle (photograph courtesy of Dr. Roger Craig, University of Massachusetts) and schematic views of (b) the muscle sarcomere and (c) the structural components of the giant protein titin. Titin Ig and FnIII domains are shown in circles and ovals, respectively. Five Ig domains and one FnIII domain with known atomic structure are labeled. One very specialized structure in a sarcomere is the giant muscle protein titin, which is the largest protein identified in nature, with a molecular weight of nearly ∼4 MDa and a contour length of ∼1 µm [1, 2]. Anchored at the Z and M lines, a string-like titin molecule spans half of the sarcomere in close contact with the thick filament along the A band, but flexible along the I band (Fig 1b and c). The major structural components of titin are bead-like β-sandwich protein modules, most notably the immunoglobulin-like domains, or Ig domains, named after immunoglobulin where the homologous structural motif was first identified. The stable core of an Ig domain is formed from strands of β-sheets, connected through flexible loops from which protrusions form binding sites for antigens in immunoglobulins. However, in titin the Ig domains are not involved in immunological recognition. Instead, they contribute to the passive stiffness of a stretched sarcomere. 3 Titin is the molecular spring in a muscle sarcomere Muscle force generation is dependent on the contractile and stretching motions that take place in the sarcomere. The contractile movement generates active force while the stretching movement generates passive force. The combination of active and passive forces within muscle fibers ultimately gives rise to the muscular force required for various physical motions, such as your heart beating, walking, or turning this page (not yet!). At the molecular level, the contractile motion is due to the sliding of myosin filaments over the actin filaments. Triggered by the release of calcium ions upon muscle excitation and powered by the hydrolysis of ATPs, a series of conformation changes occur in myosin cross-bridges that associate with actin filaments. These conformational changes induce a power stroke event that pulls the thin filament past the thick filament and towards the central M-line at a rate of up to 15 µm/s, exerting the active force. In contrast, when sarcomere is relaxed and further stretched beyond its slack length, a passive force develops to dampen the force of extension and restore the sarcomere to its resting length. An essential characteristic of striated muscle is its elasticity, a mechanical property necessary for the reversible contractile and stretching movement of sarcomere. This elasticity permits myofibrils to be stretched to twice their resting length without damaging their structure. The physiological sarcomere length ranges from 1.9 to 2.5 µm in cardiac muscle and 2.2 to 3.8 µm in soleus, a skeletal calf muscle. Investigations of myofibrils during contraction and relaxation suggest that neither actin nor myosin filaments confer the elasticity seen during force generation, since the length of both filaments appear unchanged during the contraction-extension cycle. Instead, the elastic property of sarcomere appears to arise from another set of muscular filaments, composed of the protein titin. It is the lengthening of titin molecules that generates the passive force which is crucial for maintaining the integrity of the sarcomere. Electron micrographs and gene sequence analysis reveal that the architecture of titin is built upon a linear assembly of 300 immunoglobulin-like (Ig) or fibronectin III-like (FN-III) domains, in addition to several uncharacterized segments. In the A-band or Z-line regions, titin intimately associates with proteins located in the thick and thin filaments, respectively. The only part of titin that appears to move freely is its I-band region, which provides the major contribution to passive elastic properties for the sarcomere. Titin I-band domains of variable composition, called isoforms, have been found in different types of muscle tissue, indicating that the specific distribution of domain types within the titin spring determine its stiffness and extensibility. Molecular fine tuning for the titin spring Approximately ninety percent of titin’s mass by composition lie in the globular Ig or FN-III domains (Fig 1c). Each domain consists of ∼100 residues folded into a β-sandwich motif. The titin A-band is built from highly conserved Ig or FnIII domains that are arranged in regular patterns correlating to the structural composition of associated thick filaments. The repeating patterns seen in both titin and myosin leads to the hypothesis that the titin A-band may regulate the assembly of myosin in the early stage of muscle development. In contrast, the components of titin I-band exhibit prominent passive elasticity that characterizes the spring-like property of the sarcomere. The termini of titin I-band are composed of two well conserved Ig segments, the distal (15 Ig domains) and the proximal (22 Ig domains) segments, respectively. The two segments are connected by three isoform-dependent segments: (i) a variable length Ig segment, 4 (ii) PEVK region, named due to the abundance of proline (P), glutamic acid (E), valine (V), and lysine (K), and (iii) N2 (N2A and/or N2B) regions. The PEVK and N2 regions, rich in charged residues and prolines, do not form stable secondary structures and unfold completely upon stress, elongating two to four times their folded length during muscle extension. In contrast to the flexible PEVK and N2 domains, Ig domains appear to be designed to resist extreme tension and even unfold reversibly at a higher load as the stretch in the sarcomere continues. Thus, the resistance to stress and the reversible unfolding of Ig domains provide a mechanism to prevent over-stretching of muscle. Figure 2: (a) Structure of Titin Ig domain I27, (b) and (c) Structural alignment and protein sequence com- parison of five human titin Ig domains: I1 (PDB code: 1G1C), I27 (1TIT), Z1 (1RJ0), Z2 (1RJ0), M5 (1TNM). Details of how to align these five Ig domains and reproduce the snapshot (c) with the program VMD are given in Exercise 1. The composition of a bungee cord determines how much it will stretch during a jump. For example, sheathed bungee cords typically have a rubber core surrounded by a nylon wrapping and stretch to around twice their resting length during a jump. In contrast, all rubber cords consist of many tiny strands of rubber wound together to form one solid cord, and typically stretch to four times their resting length during a jump. The elasticity of I-band titin follows a similar scheme where its composition determines whether it is stiff or elastic. Instead of rubber strands, titin is built from protein segments with different folds, and the ratio of these folds determine to what extent the sarcomere can stretch and still reversibly recover to equilibrium length without permanent damage. The complete sequence of the human titin gene contains 363 exons that potentially encode a total of 38,138 amino acid residues [3]. One third of titin exons are differentially expressed, 5 giving rise to variants with variable domain compositions. For example, the titin isoform found in the human soleus, a leg muscle, contains an extended (ten times longer) PEVK region, and four times as many Ig domains compared to the cardiac titin isoform [4]. Thus, these titin isoforms possess fine-tuned elastic responses for different types of muscle. The elastic behavior of coiled PEVK or other unique domains appear easy to understand because they do not form stable structures.
Details
-
File Typepdf
-
Upload Time-
-
Content LanguagesEnglish
-
Upload UserAnonymous/Not logged-in
-
File Pages18 Page
-
File Size-