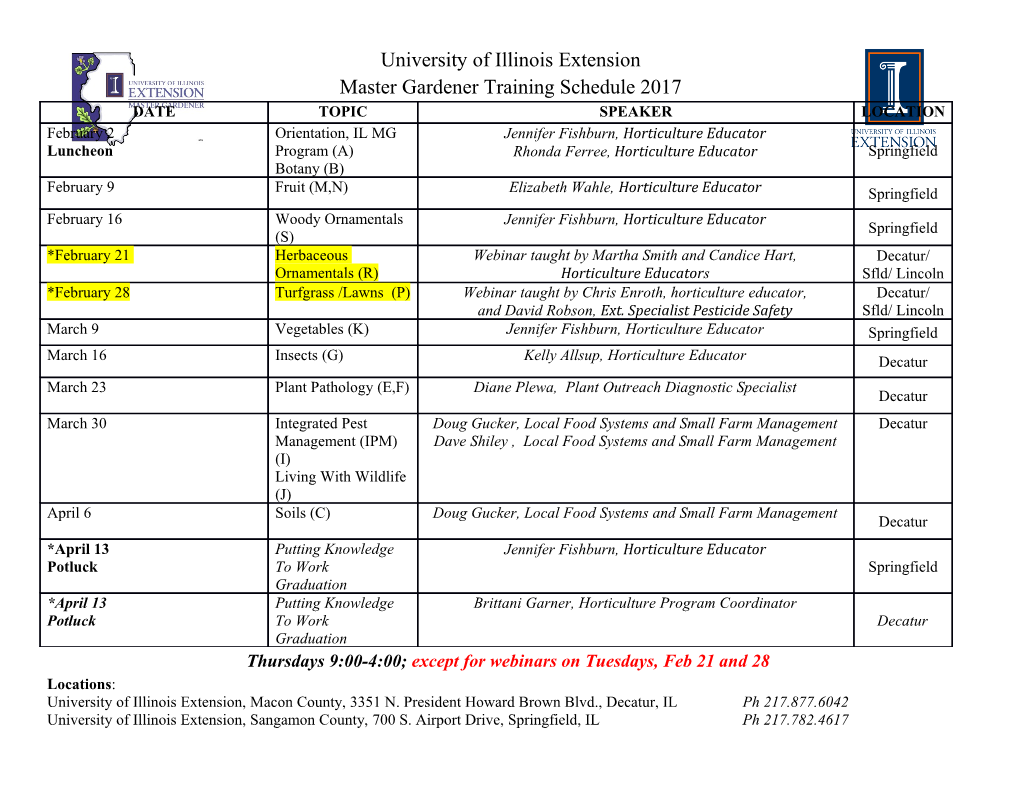
EXPERIMENTAL EVALUATION OF GLAUCONITIC SEDIMENTS FOR IN-SITU CARBON SEQUESTRATION A THESIS SUBMITTED TO THE FACULTY OF UNIVERSITY OF MINNESOTA BY TIMOTHY J. KIESEL IN PARTIAL FULFILLMENT OF THE REQUIREMENTS FOR THE DEGREE OF MASTER OF SCIENCE WILLIAM E. SEYFRIED JR. APRIL 2018 © Timothy J. Kiesel 2018 Acknowledgements I would first like to acknowledge my adviser Bill Seyfried for accepting me into the Department of Earth Sciences, for sharing his knowledge, experience, and time with an inexperienced student, and for his patience and motivation. I would also like to acknowledge the financial support and considerable laboratory space he provided for the work that allowed this thesis to be possible and the opportunity to work on an engaging project and gain invaluable experience. Special thanks go to Andrew Luhmann for his hours of invaluable instruction and expertise in experimental design and another special thank you goes to Ben Tutolo for hours of instruction and expertise in thermodynamics calculations. I wish to thank Chunyang Tan for designing and constructing experimental equipment and developing the associated control software. I thank Rick Knurr, Peter Solheid, Brian Bagley, Nick Seaton and Amy Myrbo collectively for the invaluable instrumental work that made this project possible, their expertise and the time they took to give me hands-on training. My sincere thanks go to my fellow lab mates Adam Schaen, for instructing me on the use of lab equipment and Drew Syverson, for always being available to discuss scientific concepts. I would like to acknowledge Sharon Kressler and Jennifer Petrie for helping me find my way through the paperwork to graduation. I would like to thank the faculties of the departments of Earth Sciences and at the University of Minnesota, especially my committee members Josh Feinberg and Dan Jones for their insightful comments and questions. I would also like to thank my parents Bill and Joanne for their love and support. i Abstract Flow through experiments were conducted with intact glauconite-bearing Franconian Stage sandstone cores from the Tunnel City Group to determine the effectiveness of iron carbonate precipitation as a trapping mechanism for CO2. In addition to quartz and glauconite, the rock contained small amounts of dolomite microspar. Mossbauer spectroscopy analysis indicated that the ratio of ferrous to ferric iron in the glauconite-bearing sandstone was 0.24. A key component of the experimental design entailed the use of a computer assisted fluid delivery system that permitted precise control of fluid flow rate (0.01 ml/min), as well as confining and pore fluid pressure (200 and 150 bar, respectively). Temperature was held constant at 150°C. The source fluid was a 1 molal NaCl brine charged with approximately 0.58 mol CO2/kg solution. A second experiment was carried out to test the effect of imposed reducing conditions on the formation of iron carbonate minerals and phase relations involving glauconite. Permeability was continuously monitored and observed to decrease by approximately an order of magnitude for both experiments. The dissolved chemistry of outlet fluid provided evidence of fluid saturation with dolomite and siderite, while placing constraints on rates and processes of mass transfer. Potentiometric titrations performed on fluid samples revealed a decrease in alkalinity, consistent with the precipitation of carbonate minerals. This suggests that glauconitic sandstones may be a favorable host rock for carbon sequestration. ii Table of Contents List of Tables………………………………………...…………...iv List of Figures………………………………………….………….v Introduction………………………………………………………..1 Experimental Methods………………………………………….....5 Results and Discussion…………………………………………..10 Bibliography………………………………………………….….46 Appendix………………………………………………………....53 iii List of Tables Table 1: Physical evolution of the core………………………………………........……..26 Table 2: Bulk composition of the sandstone in weight percent………………………….27 Table 3: Abundance of minerals in the core……………………………………………..28 Table 4: Chemical composition of the glauconite in weight percent………………….…29 Table 5: Dissolved chemistry of fluids following reaction with glauconite bearing core at 150°C, 200 bars (experiment 1)………………………………………………………….30 Table 6: Dissolved chemistry of fluids following reaction with a glauconite bearing core at 150C, 200 bars in the presence of an external redox buffer (experiment 2)…………………………………………………………………………………......…..31 Table 7: Mossbauer spectra for samples of the glauconitic rock………………………...32 Table 8: Extraction efficiency of selected elements for experiment 1…………………...33 Table 9: Extraction efficiency of selected elements for experiment 2………………...…34 Table A1: Maier-Kelley heat capacity Coefficients for selected oxides………………...56 Table A2: Thermodynamic values used in the Gibb’s free energy calculations………...56 Table A3: Solubility product of Glauconite at selected temperatures………………...…57 iv List of Figures Figure 1: Glauconite is structurally similar to illite……………………………………...35 Figure 2: Schematic for the flow reactor………………………………………………...36 Figure 3: Dissolved gas data from experiment 2 plotted onto a stability diagram for iron minerals…………………………………………………………………………………..37 Figure 4: Outlet fluid chemistry for both experiments…………………………………..38 Figure 5: Saturation indices for experiment 1 under oxidizing conditions………………39 Figure 6: Saturation indices for experiment 2 which included a redox buffer…………..40 Figure 7: An altered glauconite grain from experiment 1…………………………….…41 Figure 8: Secondary mineral precipitation during experiment 2………………………...42 Figure 9: Dissolution of silicates during experiment…………………………………….43 Figure 10: XRCT image of the first experimental core……………………………….…44 Figure 11 X-ray dispersive spectroscopy of iron minerals from experiment 1……….…45 v Introduction CO2 is a ubiquitous product of human industry and emissions are expected to rise as a result of increased fossil fuel usage in developing economies. CO2 tends to absorb near visible infrared radiation as part of its vibrational modes and then emitting the energy as thermal infrared radiation. This causes a warming effect on the atmosphere. Carbon dioxide is the second most abundant greenhouse gas in earth’s atmosphere and can have a residence time of 1000 years (Solomon et al. 2009). The concentration of CO2 has recently achieved a value of ~400ppm, well above the putative 280 ppm value that represents the putative pre-industrial concentration. Thus, further fossil fuel burning will have detrimental effects on global climate and the marine ecosystem. In order to reduce the effect of these emissions it has been proposed to capture carbon dioxide at a point source and then inject the CO2 into deeply buried geological formations (DePaolo and Cole, 2013). Storage may be accomplished by four different mechanisms: structural trapping, dissolution, capillary trapping and mineral formation (Kumar et al. 2005). Structural trapping is reliant on an impermeable layer of rock to limit the flow of water and supercritical CO2 similar to a cap rock in petroleum reservoirs. Solubility trapping is achieved when the carbon dioxide is injected into the formation and dissolves into the subsurface fluids (Gilfillan et al. 2009). Dissolution of CO2 into water causes hydrolysis of the compound to form carbonic acid which can lower the pH substantially. In the third method of sequestration, water contained in the pore spaces of sedimentary rock is displaced by CO2 which then is held in place by increased capillary pressure (Pentland et 1 al. 2011). Water-rock reaction with carbonic acid can alter the permeability of the rock by dissolving minerals or closing up pore throats further trapping bubbles of CO2. Mineral formation happens when cations present in the rock dissolve and react with carbonate species in the brine to form new solids. Carbonate minerals like dolomite can contain more than 40% carbon dioxide by weight. Mineralization can only account for a maximum of 18% of the CO2 trapped. Accordingly, dissolution is typically the dominant trapping mechanism (Gilfillan et al. 2009). Mineral trapping, however, is considered the most secure form of carbon sequestration because of its theoretically infinite residence time in CO2 reservoirs (IPCC 2005). To better understand this process, several studies have been carried out on a variety of host rocks and minerals. Luquot et al. 2012 carried out an experiment in which CO2 was percolated through chlorite and zeolite bearing sandstones. They reported the formation of ankerite, calcite and kaolinite from the dissolution of chamosite and laumontite. Similar experiments have been carried out on ferric sediments like banded iron formations. Sulphur dioxide was included in the experiment as a reducing agent and iron carbonates were formed as a result (Palandri et al 2009). Xu et al. (2004) suggested glauconitic sandstones could be used as a host rock for carbon fixation and modeled long term water-rock reactions in a hypothetical reservoir. Their studies considered the use of simple organic acids and sulphur dioxide as reducing agents to better simulate the conditions found in oil basins and model time scales on the order of 100,000 years. Xu et al. (2004) model predicts that glauconite will react with CO2 in coexisting brines, forming siderite from available ferrous iron. Models using 2 annite (an iron rich endmember of the biotite group) as a proxy for glauconite were run by other groups (Gunter et al. 1997), but this is not
Details
-
File Typepdf
-
Upload Time-
-
Content LanguagesEnglish
-
Upload UserAnonymous/Not logged-in
-
File Pages64 Page
-
File Size-