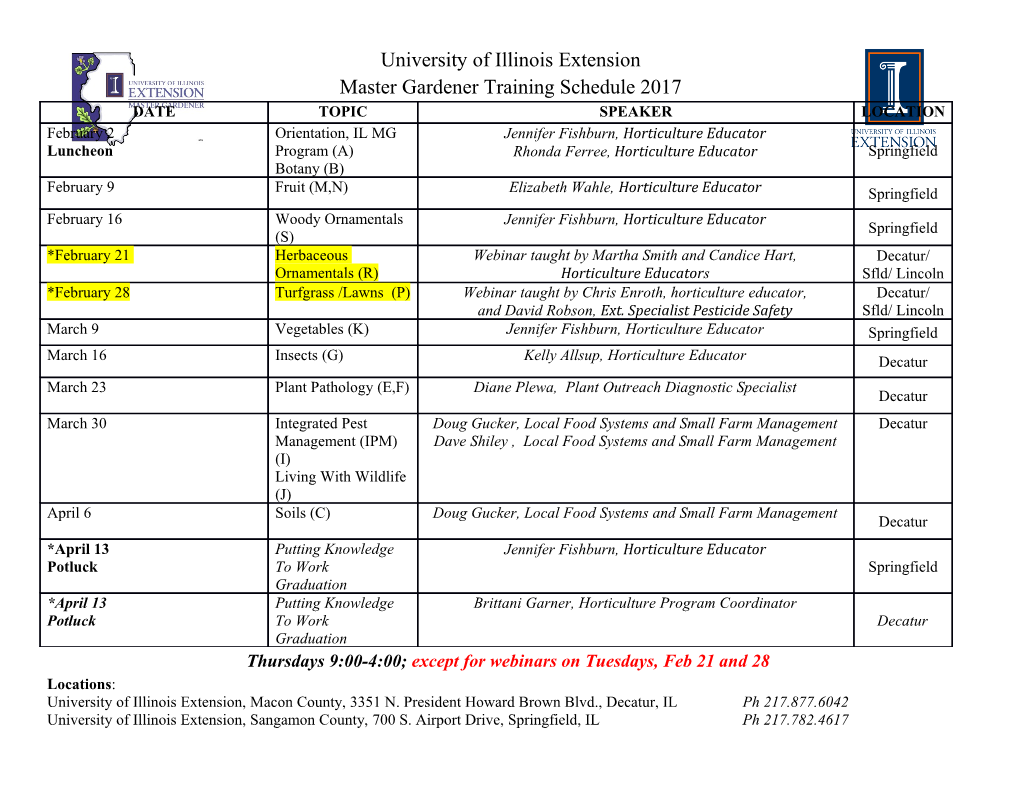
arXiv: 1308.3047 M. Titov. Perspectives of MPGD Technologies for Future Physics Projects 1 PERSPECTIVES OF MICRO-PATTERN GASEOUS DETECTOR TECHNOLOGIES FOR FUTURE PHYSICS PROJECTS1 Maxim Titov CEA Saclay, IRFU/SPP, 91191, Gif sur Yvette, France A centenary after the invention of the basic principle of gas amplification, gaseous detectors - are still the first choice whenever the large area coverage with low material budget is required. Advances in photolithography and micro- processing techniques in the chip industry during the past two decades triggered a major transition in the field of gas detectors from wire structures to Micro-Pattern Gas Detector (MPGD) concepts, revolutionizing cell-size limitations for many gas detector applications. The high radiation resistance and excellent spatial and time resolution make them an invaluable tool to confront future detector challenges at the frontiers of research. The design of the new micro-pattern devices appears suitable for industrial production. In 2008, the RD51 collaboration at CERN has been established to further advance technological developments of MPGDs and associated electronic-readout systems, for applications in basic and applied research. This review provides an overview of the state-of-the-art of the MPGD technologies and summarizes recent activities for the next generation of colliders within the framework of the RD51 collaboration. 1. INTRODUCTION Future accelerator facilities are an indispensable step towards precision determination of the Standard Model parameters, constraining of the potential new physics contributions, or, alternatively precision New Physics studies, given LHC yield a hint of new physics presence. The quest for Higgs boson discovery with the multipurpose ATLAS and CMS detectors at the LHC, imposed major detector instrumentation work, and pulled most of the technologies to their performance limits. With many fundamental physics questions within the experimental reach (e.g., precision studies of the Higgs boson couplings and other new particles discovered at the LHC) at the future accelerator facilities, a large R&D effort is needed to develop innovative concepts for radiation detection. Challenges for novel technologies include improved spatial resolution and segmentation, speed and radiation hardness while minimizing power and cost. Advances on all fronts are needed; these include modern solid state vertex and tracking detectors, gaseous detectors, crystals, cryogenic liquids, readout electronics, services (power, cooling, support and material budget), and trigger and data acquisition systems. Applications are directed at the energy, intensity and cosmic scientific frontiers, with distinct challenges, requirements and solutions: 1 Proceedings of the CMS Workshop “Perspectives on Physics and on CMS at Very High Luminosity, HL-LHC", Alushta, Ukraine, May 28-31, 2012 2 arXiv: 1308.3047 M. Titov. Perspectives of MPGD Technologies for Future Physics Projects the luminosity upgrade of the Large Hadron Collider (sLHC), the future Linear Collider (ILC or CLIC), the super high luminosity B-factory (the Belle-II), neutrino experiments and direct dark matter searches, ground-based particle astrophysics and space experiments. Improvements in detector technology often come from capitalizing on industrial progress. Integration advances in microelectronics and mechanics have resulted in novel, ever more complicated instrumentation. For example, bump bonding technology enabled low capacity connections. The possibility of producing micro-structured semi-conductor devices (with a feature size of 10 μm) and corresponding highly integrated readout electronics led to the success of pixel detectors to achieve unprecedented space-point resolution. Industrial advances in photo-lithography, microelectronics and printed circuits have opened the road for production of micro-structured gas amplification devices. During the evolution, many novel MPGD structures have arisen from the initial ideas, in general using modern photo-lithographic processes on thin insulating supports. Two designs have emerged, because of ease of manufacturing, operational stability and superior performances for tracking applications: the Gas Electron Multiplier (GEM) [1] and Micro- Mesh Gaseous Structure (Micromegas) [2]. By using pitch size of a few hundred microns, an order of magnitude improvement in granularity over wire chambers, both devices exhibit intrinsic high rate capability (>106 Hz/mm2), excellent spatial and multi-track resolution (~ 30 μm and ~ 500 μm, respectively), and single photo-electron time resolution in the ns range. Recent aging studies revealed that they might be even less vulnerable to the radiation-damage effects, compared to standard silicon micro-strip detectors, if reasonable precautions are taken on the components quality [3]. Within the broad family of MPGDs, more coarse macro- patterned detectors (e.g., thick-GEMs (THGEM) [4,5] or patterned resistive thick GEM devices (RETGEM) [6]) could offer an interesting economic solution for large-area RICH devices: namely good spatial and time resolutions, large gains (single photo-electron sensitivity), relatively low mass and easy construction - thanks to the intrinsic robustness of the PCB electrodes. Fig. 1: Historical roadmap of the MPGD technology developments. Coupling of the microelectronics industry and advanced PCB technology has been very important for the development of modern gas detectors with increasingly smaller pitch size. Usually, charge signals in MPGDs are collected on segmented electrodes (strips or pads) and amplified/recorded by external electronics. An elegant solution, that can yield a truly 3D arXiv: 1308.3047 M. Titov. Perspectives of MPGD Technologies for Future Physics Projects 3 track reconstruction, is the use of the CMOS Application Specific Integrated Circuit (ASIC), assembled directly below the Micromegas or GEM amplification structures [7]-[9]. Modern wafer post-processing technology allows for the integration of a Micromegas grid directly on top of a high-granularity (55 μm pitch) Medipix2 or Timepix chip, thus forming an integrated readout of gaseous detector (“InGrid”) [10]. Using this approach, MPGD-based detectors can reach the level of integration, compactness and resolving power typical of solid-state pixel devices. The historical roadmap for several most advanced MPGD technologies is shown in Fig. 1. The interest in the development and use of the novel micro-pattern gas detectors has led to the establishment in 2008 of the international research collaboration RD51 at CERN [11]. 2. THE “MPGD” FAMILY The modern MPGD structures can be grouped in two large families: strip and hole-type structures and micromesh-based detectors. The strip and hole-type structures are: Micro-Strip Gas Chambers (MSGC), GEM, THGEM, RETGEM, Micro-Hole and Strip Plate (MHSP), and Micro-Pixel Gas Chamber (μ-PIC). The micromesh-based structures include: Micromegas, “Bulk” Micromegas, “Microbulk” Micromegas and “InGrid”. Fig. 2: (left) Schematic view and typical dimensions of the hole structure in the GEM amplification cell. Electric field lines (solid) and equipotentials (dashed) are shown16; (right) schematic view of the triple-GEM detector. Fig. 3: (left) Electric field lines in Micromegas; (rigth) schematic drawing of the Micromegas detector. Introduced in 1996, a Gas Electron Multiplier (GEM) detector consists of a thin-foil 4 arXiv: 1308.3047 M. Titov. Perspectives of MPGD Technologies for Future Physics Projects copper-insulator-copper sandwich chemically perforated to obtain a high density of holes. The GEM manufacturing method, developed at CERN, is a refinement of the double-side printed circuit technology. Due to the simultaneous etching from both sides of the foil, the holes acquire a double-conical cross-section. The hole diameter is typically between 25 μm and 150 μm, while the corresponding distance between holes varies between 50 μm and 200 μm. The central insulator is usually (in original design) the polymer kapton, with a thickness of 50 μm. Application of a potential difference between the two sides of the GEM generates the electric field indicated in Fig. 2(left). Each hole acts as an independent proportional counter. Electrons released by the primary ionization particle in the upper drift region (above the GEM foil) are drawn into the holes, where charge multiplication occurs in the high electric field (50–70 kV/cm). Most of avalanche electrons are transferred into the gap below the GEM. Several GEM foils can be placed at short distances (typically 1–2 mm) to distribute the gas amplification among several stages (see Fig. 2(right)). A unique property of GEM detector is a full decoupling of the amplification stage (GEM) and the charge collection electrode - readout board of arbitrary pattern, placed below the last GEM. The signal detected on the PCB is generated only by the avalanche electrons (the ions do not contribute to the signal) and is typically a few tens of nanosecond for 1 mm-wide induction gap. Introduced in 1996, the Micromegas is a thin parallel-plate avalanche counter, as shown in Fig. 3. It consists of a few mm drift region and a narrow multiplication gap (25–150 μm) between a thin metal grid (micromesh) and the readout electrode (strips or pads of conductor printed on an insulator board). Regularly spaced supports (insulating pillars) guarantee the uniformity of the gap between the anode plane and the micromesh, at the expense of a small, localized loss of efficiency. A
Details
-
File Typepdf
-
Upload Time-
-
Content LanguagesEnglish
-
Upload UserAnonymous/Not logged-in
-
File Pages14 Page
-
File Size-