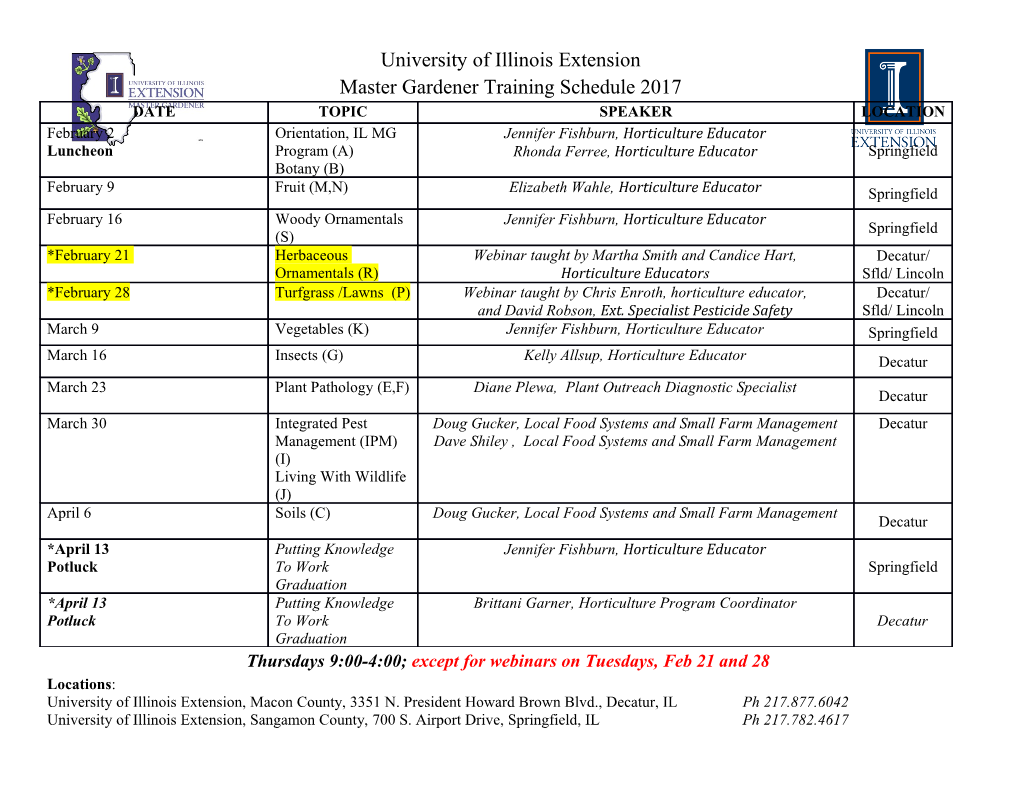
AperTO - Archivio Istituzionale Open Access dell'Università di Torino Surface Water Photochemistry This is the author's manuscript Original Citation: Availability: This version is available http://hdl.handle.net/2318/1622297 since 2017-01-18T17:16:36Z Publisher: Royal Society of Chemistry Terms of use: Open Access Anyone can freely access the full text of works made available as "Open Access". Works made available under a Creative Commons license can be used according to the terms and conditions of said license. Use of all other works requires consent of the right holder (author or publisher) if not exempted from copyright protection by the applicable law. (Article begins on next page) 08 October 2021 This is the author's final version of the contribution published as: Paola Calza; Davide Vione. Surface Water Photochemistry. Royal Society of Chemistry. 2016. pp: 1-297. When citing, please refer to the published version. Link to this full text: http://hdl.handle.net/2318/1622297 This full text was downloaded from iris - AperTO: https://iris.unito.it/ iris - AperTO University of Turin’s Institutional Research Information System and Open Access Institutional Repository Surface Water Photochemistry Chapter 1 Introduction Davide Vione* and Paola Calza Department of Chemistry University of Torino Via P. Giuria 5 10125 Torino Italy [email protected] 1. Introduction In the following sections of this introductory chapter, some basic pieces of information are provided that will be useful for the understanding of the chapters that follow. 1.1. Basic principles of photophysics and environmental photochemistry 1,2 In the natural environment sunlight is the source of radiation ( λ > 280 nm) and, for photochemistry to be operational, one needs molecules that absorb radiation above 280 nm. When a molecule absorbs a photon, an electron is excited from the ground state (often it is the ground state from both an electronic and a vibrational point of view) to a vibrationally excited state of an electronically excited state. In the case of organic molecules, the ground electronic state is usually a singlet one (S 0), where the electrons are all paired in full orbitals with antiparallel spin. When the molecule absorbs radiation, it reaches an excited state that is also a singlet one. It could be the first (S 1), the second (S 2) or a higher excited singlet state depending on the energy of the photon and of the molecular states (see Figure 1). To begin with, assume that the electron reaches a vibrationally excited state of S 1. In some cases there might be an excess of vibrational energy, which may cause an excessive strain on the weakest molecular bond that is involved in the vibrational motions triggered by radiation absorption. In this case, bond breaking would take place: indeed, the very word photolysis suggests the occurrence of photoinduced bond breaking. As an alternative, the molecule can undergo fast vibrational deactivation to the S 1 ground state. From here, the molecule could take part in chemical reaction, thermal or photochemical deactivation to S 0, or inter-system crossing. Chemical reaction is quite rare in the case of the short-lived excited singlet states, but it accounts for instance for the photoisomerisation of 2-chlorophenol to 5-ring species. 3 Thermal deactivation (internal conversion) can be of some importance in the field of environmental photochemistry, because it would be enhanced by inter-molecular interactions that could for instance account for the limited photophysical activity and photochemical reactivity of high- molecular weight chromophoric dissolved organic matter (HMW CDOM). 4 Place figure 1.1 near here In some cases, the S 1 → S 0 transition takes place by emission of a fluorescence photon. If this is the case, the electron reaches an excited vibrational state of S 0 and then the ground one by vibrational relaxation. The double loss of vibrational energy, in both S 1 and S 0, explains why the wavelength of the emitted (fluorescence) photon is higher (and, therefore, the associated energy is lower) 2 compared to the absorbed photon. Finally, the electron in the S 1 state can undergo inter-system crossing (ISC) to the first excited triplet state (T 1). The ISC implies spin inversion, and a further inversion would be required for the transition from T 1 to S 0. Such a transition is formally forbidden by the selection rules of quantum mechanics, which in practice means that its probability is low and that the T 1 state is longer-lived than S 1. The quite long lifetime means that T 1 can undergo chemical reactions, for instance with the solvent or with dissolved molecules, as well as intra-molecular rearrangements. A common energy-transfer reaction takes place with dissolved O2, which is favoured by the fact that the O 2 ground state is a triplet one. The reaction yields singlet oxygen 1 ( O2), while the molecule usually reaches the S 0 state. Internal conversion from T 1 to S 0 is also possible, in which case the energy is thermally lost through e.g. collisions with the solvent. In some rare cases, the transition from T 1 to S 0 can take place by emission of a phosphorescence photon. Similarly to the case of fluorescence emission, in the case of phosphorescence the molecule reaches a vibrationally excited state of S 0 from which a further vibrational loss of energy takes place. Upon radiation absorption, other excited states different from S 1 may be reached. If the energy is high enough (which is more common in the case of the environmentally non-relevant UVC radiation, λ < 280 nm), the electron may be abstracted from the molecule to produce its photoionisation. 5 Interestingly, the irradiation of CDOM is well known to yield aquated electrons. 3,4 If the photon energy is not high enough to cause photoionisation, higher excited states than S 1 may be reached (S 2, S 3 and so on). In the case of most organic molecules, the electron reaches a vibrationally excited state of the singlet state S n (n > 1), from which it undergoes vibrational deactivation to the ground vibrational level of S 1. This is quite important for photochemistry and photophysics because, independently of the excitation wavelength, the molecule always reaches the ground state of S 1, from which its further evolution will be the same independently of the details of the excitation process. It is the so-called Kasha’s rule, according to which for instance the fluorescence emission wavelength is always the same (following the S 1 → S 0 transition) independently of the excitation wavelength (which may cause transitions of the kind S 0 → S 1, S 0 → S2 and so on, depending on the photon energy). An interesting application of the Kasha’s rule can be seen in the case of the irradiation of anthraquinone-2-sulphonate (AQ2S). This molecule is not fluorescent (its ISC is much more efficient than the energy deactivation by fluorescence emission), but upon irradiation AQ2S forms fluorescent hydroxyderivatives. 6 The fluorescence excitation-emission matrix spectrum of irradiated AQ2S is reported in Figure 2, showing a single emission band (in the range of 550-600 nm) that, however, corresponds to several excitation bands at different wavelengths, ranging from 200 to 500 nm and suggesting various S 0 → S n transitions. In some cases, the Kasha’s rule is apparently not followed. This is the case for instance of the fluorescence spectrum of 4-phenoxyphenol, which has four paired emission bands (see Figure 3). One pair has emission at 300-350 nm, which corresponds to two excitation bands at 200-250 and 250-300 nm. The second pair has emission at 350-425 nm, corresponding to two excitation bands around 250 and 300 nm. According to Kasha’s rule one would expect a single emission wavelength 3 (S 1 → S 0 transition), linked to the occurrence of one pair only or to four bands that should be vertically placed as in the case of Figure 2. However, 4-phenoxyphenol exists in aqueous solution in three different rotational conformations that undergo very slow inter-conversion (compared to the typical fluorescence lifetimes). It has been shown that a conformer accounts for the bands with emission at 300-350 nm, the other two for the bands with emission at 350-425 nm. 7 Place figure 1.2. near here Place figure 1.3. near here 1.2. Photosensitisers and photoinduced transients in surface waters Several photochemical processes can take place in sunlit surface waters. Some of them involve the absorption of sunlight by the molecule(s) that are transformed, following reaction pathways that have been partly described in section 1.2 and that will be more extensively dealt with in Chapter 4. 3,8,9 However, additional reactions are triggered by the absorption of sunlight photons by naturally occurring photoreactive species called photosensitisers. Among these naturally compounds there are 10 chromophoric dissolved organic matter (CDOM), nitrate, nitrite, Fe species and H 2O2. The (photo)chemistry of Fe, which also partially involves that of H 2O2, is quite complex and will be the subject of a separate chapter. The present introduction will provide a general overview of the photochemistry of CDOM, nitrate and nitrite, to be further elucidated later on in this book. CDOM is, almost beyond any doubt, the single most important photosensitiser (or better, class of sensitisers) that occurs in natural waters. Its complex and not yet completely elucidated molecular structure has been the subject of intense debate. Today, many scientists accept that it could be a supra-molecular (rather than a macromolecular) combination of smaller compounds, which form aggregates that vary for the apparent molecular size.
Details
-
File Typepdf
-
Upload Time-
-
Content LanguagesEnglish
-
Upload UserAnonymous/Not logged-in
-
File Pages365 Page
-
File Size-