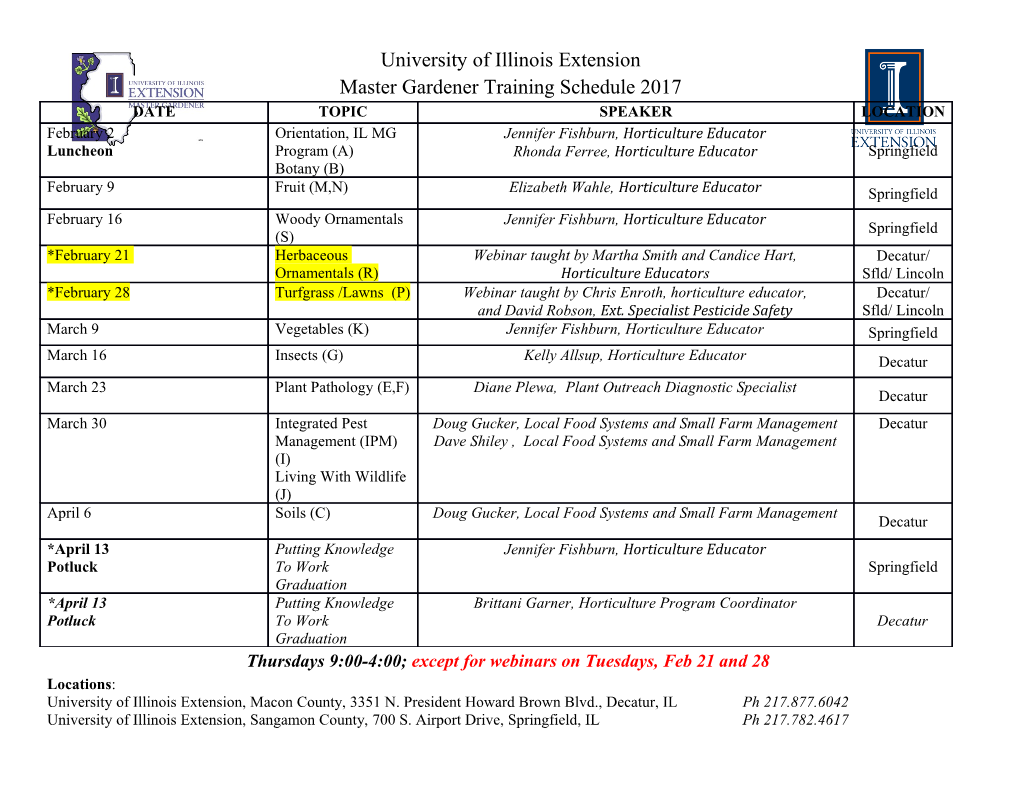
(Galerkin) Finite element approximations The finite element method (FEM): special choice for the shape functions φ. ˜ Ne = 5 Subdivide Ω into elements Ωe: Ne Ω = Ωe e=1 [ Ω1 Ω2 Ω3 Ω4 Ω5 Ωe1 Ωe2 = x = a x = b ∩ ∅ Approximate u on each element separately by a polynomial of some degree p, for example by Lagrangian interpolation (using p + 1 nodal points per element). The end points of an element must be nodal points. Example: linear elements Global shape functions: Ne = 5 n T 1 uh(x) = uiφi(x) = φ (x)u φ3(x) i=1 ˜ ˜ X Ω1 Ω2 Ω3 Ω4 Ω5 n: number of global nodal points. x1 = ax2 x3 x4 x5 x6 = b Local element shape functions: e e e T e u (x) = u φ1(x)+u φ2(x) = φ (x)u h 1 2 ˜ φ1(x) φ2(x) ˜ 1 1 T with φ = [φ1(x), φ2(x)] and ˜ e e x x2 x x1 e e e e φ1(x) = − , φ2(x) = − x1 x2 x1 x2 xe xe xe xe 1 − 2 2 − 1 Example: quadratic elements Local element shape functions: φ1(x) φ2(x) φ3(x) 1 1 1 e e e e e e e e e x1 x2 x3 x1 x2 x3 x1 x2 x3 e e e T e uh(x) = u1φ1(x) + u2φ2(x) = φ (x)u ˜ ˜ T with φ = [φ1(x), φ2(x), φ3(x)] and ˜ (x xe)(x xe) (x xe)(x xe) φ (x) = − 2 − 3 , φ (x) = − 1 − 3 , 1 (xe xe)(xe xe) 2 (xe xe)(xe xe) 1 − 2 1 − 3 2 − 1 2 − 3 (x xe)(x xe) φ (x) = − 1 − 2 3 (xe xe)(xe xe) 3 − 1 3 − 2 Example: higher-order elements General polynomials of order p: p+1 e e T e uh(x) = ui φi(x) = φ (x)u i=1 ˜ ˜ X T with φ = [φ1, φ2, . , φp+1]. ˜ Various expansions possible: B Gauss-Lobatto integration points (includes end points) and Lagrangian interpolation (Spectral elements). B Hierarchical base functions: end points are nodes but internal shape functions have no nodes (similar to Legrende polynomials). (hp-FEM). B Legrendre polynomials in discontinuous Galerkin methods. Global numbering 4 N =3 e uh(x) T uh(x) = uiφi(x) = φ (x)u i=1 ˜ ˜ X u 2 u3 u1 u4 u1 φ1(x) u φ (x) Ω1 Ω2 Ω3 u = 2 , φ(x) = 2 u φ (x) x1 = ax2 x3 x4 = b ˜ 3 ˜ 3 u φ (x) 1 4 4 φ (x) Ne = 3 3 Ω1 Ω2 Ω3 x1 = ax2 x3 x4 = b Local numbering in elements Weak form: Find uh in Sh such that dv du ( h,A h) + v (b)h = (v , f) for all v V dx dx h b h h ∈ h Split: N N e dv du e ( h,A h) + v (b)h = (v , f) for all v V dx dx h b h h ∈ h e=1 e=1 X X Write in each element e: 2 2 e e T e e T uh(x) = ui φi(x) = φ (x)ue, vh(x) = vi φi(x) = φ (x)ve i=1 ˜ ˜ i=1 ˜ ˜ X X T e e T where ue = (u1, u2) and φ (x) = (φ1(x), φ2(x)). ˜ ˜ Element matrix and vector We get: Ne Ne T T (ve Keue) + vnhb = ve f e e=1 ˜ ¯ ˜ e=1 ˜ ˜ X X where dφ dφT dφ dφT Ke = ˜,A ˜ = ˜A ˜ dx ¯ dx dx e Ω dx dx Z e f e = (φ, f)e = φf dx ˜ ˜ ZΩe ˜ are the element matrix Ke and element vector f e. ¯ ˜ Local global (assembling) → The local vectors ue and ve are part of the global vectors: ˜ ˜ ue = P eu, ve = P ev, ˜ ¯ ˜ ˜ ¯ ˜ So we get Ne Ne Ne T T T T ˜ v Keue = v P KeP e u = v Ke u ˜e ¯ ˜ ˜ ¯ e ¯ ¯ ˜ ˜ ¯ ˜ e=1 e=1 ˜ e=1 X X Ke X ¯ Ne Ne | {z } Ne vT f = vT P T f = vT f˜ e e e e e e=1 ˜ ˜ e=1 ˜ ¯ ˜ ˜ e=1 ˜ X X f˜ X e | ˜{z } Weak form Substitution into the weak form: vT Ku = vT f for all v ˜ ¯ ˜ ˜ ˜ ˜ or Ku = f ¯ ˜ with ˜ Ne ˜ K = Ke ¯ e=1 ¯ X 0 Ne 0 f = f˜ + . e ˜ e=1 ˜ 0 X h − b Example (1) For example: u1 u2 0 1 0 0 u2 u2 = = = P 2u u3 0 0 1 0 u3 ¯ ˜ ˜ u4 P 2 ¯ 0| 0 {z } 2 2 ˜ T 1 0 K11 K12 0 1 0 0 K2 = P 2 K2P 2 = 2 2 ¯ ¯ ¯ ¯ 0 1 K21 K22 0 0 1 0 0 0 0 0 0 0 2 2 0 K11 K12 0 = 2 2 0 K21 K22 0 0 0 0 0 Example (2) Assembly of K: ¯ 1 1 K11 K12 0 0 0 0 0 0 0 0 0 0 1 1 2 2 K21 K22 0 0 0 K11 K12 0 0 0 0 0 K = + 2 2 + 3 3 ¯ 0 0 0 0 0 K21 K22 0 0 0 K11 K12 0 0 0 0 0 0 0 0 0 0 K3 K3 21 22 1 1 K11 K12 0 0 1 1 2 2 K21 K22 + K11 K12 0 = 2 2 3 3 0 K21 K22 + K11 K12 0 0 K3 K3 21 22 Similar for assembly of f. ˜ Exercise 5 The ‘bandwidth’ of K for linear shape functions is 3. How large is the bandwidth for K when using polynomial¯ shape functions of order k, k 1? ¯ ≥ Dirichlet conditions Renumber and split vector and matrix (‘u’ unknown, ‘p’ prescribed) uu vu Kuu Kup f u u = ˜ , v = ˜ ,K = ¯ ¯ , f = ˜ ˜ up ˜ vp ¯ Kpu Kpp ˜ f p ˜ ˜ ¯ ¯ ˜ Note: up prescribed values, vp = 0. Weak form ˜ ˜ ˜ vT Ku = vT f for all v ˜ ¯ ˜ ˜ ˜ ˜ with vp = 0 leads to ˜ ˜ T T vu (Kuuuu + Kupup) = vu f u for all vu ˜ ¯ ˜ ¯ ˜ ˜ ˜ ˜ or Kuuuu = f u Kupup ¯ ˜ ˜ − ¯ ˜ 1D convection-diffusion-reaction equation 1D convection-diffusion-reaction Eq.: find u(x) such that for x (a, b) ∈ ∂u ∂u ∂ ∂u + a (A ) + bu = f ∂t ∂x − ∂x ∂x and u = ua(t), at x = a, t > 0 (ΓD) du A = h (t) at x = b, t > 0 (Γ ) − dx b N Notes: B Strong form; Classical (strong) solution u(x, t) 0 2 B f(x, t) C (a, b) (continuous) then u C (a, b) (twice continuously differentiable)∈ ∈ Oldroyd-B/UCM viscoelastic model λ τ5 +τ = 2ηD where τ5= τ˙ L τ τ LT − · − · or ∂τ τ η + ~u τ L τ τ LT + = 2 D ∂t · ∇ − · − · λ λ ∂u ∂u bu f ∂t a∂x |{z} | {z } | {z } | {z } Diffusion is missing (A = 0). Dimensionless form (1) Scaling: t = tct∗ tc : characteristic time u = Uu∗ U : characteristic value solution x = Lx∗ L : characteristic length scale Dimensionless variables: (1). O 2 U ∂u∗ aU ∂u∗ AU ∂ u∗ + 2 2 + bUu∗ = f tc ∂t∗ L ∂x∗ − L ∂x∗ Relative to convection: 2 L ∂u∗ ∂u∗ 1 ∂ u∗ bL L + 2 + u∗ = f atc ∂t∗ ∂x∗ − Pe∂x∗ a aU aL Pe = : P´eclet number, convection/diffusion. A Dimensionless form (2) Time scales: L convection: B a L2 diffusion: B A 1 source: (’relaxation time’) B b B time scales in b.c. B externally or internally generated frequencies (’von Karman vortex’) We choose tc = L/a (convection) and get 2 ∂u∗ ∂u∗ 1 ∂ u∗ + 2 + b∗u∗ = f ∗ ∂t∗ ∂x∗ − Pe∂x∗ bL L with b = and f = f. Pe > 1 : convection dominated ∗ a ∗ aU Exercise 6 L2 Assume b = 0 and f = 0. We take for the typical time scale t = (diffusion c A time scale). Show that we now have the non-dimensional form 2 ∂u∗ ∂u∗ ∂ u∗ + Pe 2 = 0 ∂t∗ ∂x∗ − ∂x∗ When will this non-dimensional form be preferable over the one on the previous slide? Steady state 1D convection-diffusion-reaction equation steady state: ∂u/∂t = 0 du d du (u) = a (A ) + bu = f L dx − dx dx : linear operator, with b.c. L u = ua, at x = a (ΓD) du A = h at x = b (Γ ) − dx b N Weak form Multiply with test function v and integrate: (v, u f) = 0 for all v L − Partial integration of the diffusion term and inserting b.c. we get: Find u S such that ∈ dv du du ( ,A ) + (v, a ) + (v, bu) + v(b)h = (v, f) for all v V dx dx dx b ∈ where S and V are appropriate spaces. Galerkin FEM approximations (1) Approximation spaces Sh and Vh: n T uh(x) = uiφi(x) = φ (x)u i=1 ˜ ˜ X n T vh(x) = viφi(x) = φ (x)v i=1 ˜ ˜ X where φ(x) are global shape functions. Substituting this into the weak form gives: Find u˜ S such that h ∈ h dv du du ( h,A h) + (v , a h) + (v , bu ) + v (b)h = (v , f) for all v V dx dx h dx h h h b h h ∈ h or vT Ku = vT f for all v ˜ ¯ ˜ ˜ ˜ ˜ Galerkin FEM approximations (2) and thus Ku = f ¯ ˜ ˜ with T dφ dφ dφi dφj K = ( ˜,A ˜ ) or Kij = ( ,A ) ¯ dx dx dx dx T dφ dφj + (φ, a ˜ ) + (φi, a ) ˜ dx dx T + (φ, bφ ) + (φi, bφj) ˜ ˜ f = (φ, f) hbφ(b) fi = (φi, f) hbφi(b) ˜ ˜ − ˜ − Galerkin FEM approximations (3) Build from element matrices. For example with constant coefficients and linear element shape functions: dφ dφT 1 1 1 ( , )e = − “stiffness matrix” dx˜ dx˜ h 1 1 − dφT 1 1 1 (φ, )e = − “convection matrix” dx˜ 2 1 1 ˜ − h 2 1 (φ, φT ) = “mass matrix” e 6 1 2 ˜ ˜ where h is the element length.
Details
-
File Typepdf
-
Upload Time-
-
Content LanguagesEnglish
-
Upload UserAnonymous/Not logged-in
-
File Pages93 Page
-
File Size-