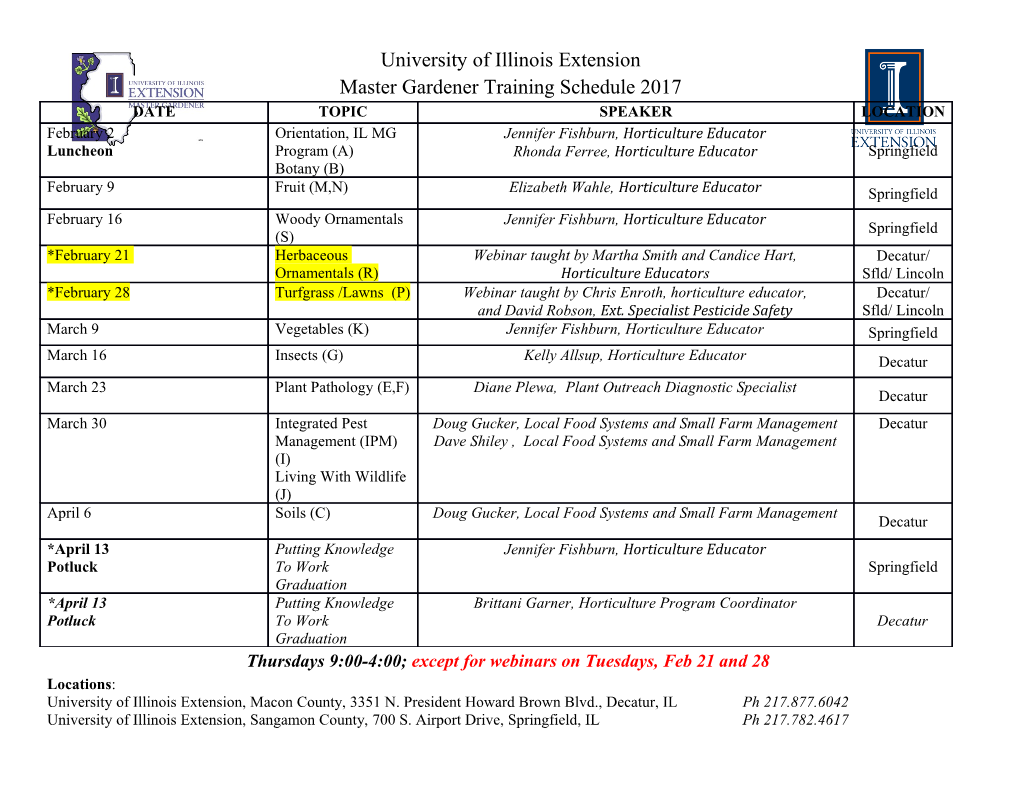
FLEXIBLE WATER TREATMENT BY ELECTROCOAGULATION (EC) AND ION CONCENTRATION POLARIZATION (ICP) HYBRID SYSTEM Siwon Choi1 and Jongyoon Han2,3* 1Department of Chemical Engineering, 2Department of Electrical Engineering and Computer Science, 3Department of Biological Engineering, Massachusetts Institute of Technology, USA ABSTRACT Conventional water treatment process is composed of desalination (salt removal) and pre/post- treatment of desalination to remove particles, chemicals, and other potential foulants for desalination. In this work, we developed a microfluidic proof-of-concept for total water treatment, which removes salt ions and small colloidal particles, by combining ion concentration polarization desalination and electrocoagulation. We demonstrated a continuous EC-ICP operation to remove salt and suspended solids. We also demonstrated that our system is flexible in terms of the type and concentration of contaminants it can handle. Our system will find applications as a small scale water treatment system. KEYWORDS: Electrocoagulation, Ion concentration polarization (ICP), Water treatment, Desalination INTRODUCTION Electrocoagulation (EC) is an electrochemical water treatment method, in which the metal ions, released from a sacrificial metal anode from electrolytic reaction, act as coagulants to destabilize pollutant particles. The resulting floc of pollutants can be easily removed by physical separation methods, such as sedimentation or flotation (Figure 1A). EC has been used to remove various pollutants, including organics, oil, suspended solids, heavy metals, fluorides, and biological contaminants, with the exception of salt ions [1],[2]. Ion concentration polarization (ICP) is an electrochemical phenomenon commonly found around ion-selective membranes or electrodes. Previously, we utilized ICP to develop a desalination platform [3] (Figure 1B). Here, we incorporated EC into an ICP system by replacing the anode with a sacrificial metal anode (Figure 1C) and created an EC-ICP hybrid system that can address virtually all type of contaminants in water. Figure 1: Schematics of (A) electrocoagulation (EC), (B) ion concentration polariza- tion (ICP) desalination, and (C) EC-ICP hybrid. The hybrid system was visualized under microscope. For ease of visualization, the system was simplified to a single cell, consisting of a metal electrode and CEM on each side of the cell. A coagulate layer in white and a depletion layer with dark vortexes are shown on the surface of the anode and the CEM, respectively. The feed solution consisted of silica in 10 mM NaCl. EXPERIMENTAL The device consists of an electrolytic cell with a cationic exchange membrane(s) (CEM) that separate(s) the solutions in contact with the anode and cathode. Through this CEM, only cations can be transported according to the direction of the electric field applied, and an ion depletion zone is created (see Figure 1B). The sacrificial anode is composed of aluminum. The method for device fabrication is as 978-0-9798064-7-6/µTAS 2014/$20©14CBMS-0001 1470 18th International Conference on Miniaturized Systems for Chemistry and Life Sciences October 26-30, 2014, San Antonio, Texas, USA described in Kwak et al.[4]. The feed solution consists of 1000 mg/L colloidal silica (Davg = 22 nm) or 6.5x108 cells/mL Escherichia coli (E. coli) in various salinity. A negatively charge dye was added as a minority charge carrier in order to track the movement of ions, and the process was observed under fluorescent microscope. The silica, E. coli, and salt removal were quantified by silicomolybdate method with bicarbonate digestion [5], optical density measurements at λ=600nm (OD600) using ultraviolet- visible spectroscopy, and conductivity measurements, respectively. In demonstration of a multi-cell, continuous EC-ICP system on a microfluidic platform, a coagulation reservoir was added between EC and ICP steps to allow a longer reaction time for complete coagulation and sedimentation of particulates. RESULTS AND DISCUSSION A single cell EC-ICP process was visualized under microscope as shown in Figure 1C. The trajectory of a coagulate is tracked with the green circle on the images. The coagulates can be seen under fluorescence because the dye particles, along with silica, were trapped in the coagulates. Although most of the coagulates exited the cell, some coagulates fouled the channel near the anode. We modified the single cell EC-ICP to enhance the quality and throughput of the treated water and developed a continuous multi-cell EC-ICP system. In the new system, shown in Figure 2, the feed water is first treated with EC, where small, colloidal particles are removed from the solution; the product of EC, which contains mostly salt ions, is then desalinated by ICP. In this experiment, we chose silica to model suspended solids in 10mM NaCl. The recovery of clean water was 45% of the initial feed. As described in Table 1, silica removal was >99%, and salt removal increased linearly with the applied current. We can further increase the salt removal by increasing the channel length or current, or decreasing the recovery ratio or the flow velocity in the ICP cells. Figure 2: Continuous multi- cell EC-ICP. All solution undergoes EC treatment first, where small particu- lates are coagulated and removed. The product of EC is split into multiple ICP cells because ICP desalina- tion requires much slower flow. The process was ob- served under microscope (microscopic image of the boxed area shown on the right). Table 1. Performance of continuous, multi-cell EC-ICP. Flowrates: 800 µL/min (vavg=1m/min) in EC cell, and 120µL/min (vavg=0.25m/min) each outlet (brine and desalted) of ICP cell. Current density [A/m2] 100 200 Silica removal > 99% > 99% Salt removal (reduction in conductivity) 10% 20% Electrical energy consumption [Wh/L] 0.35 1.4 To demonstrate that our system can be applied in a wide range of salinity, we performed electrocoagulation at three different salinity: 10mM, 500mM (seawater), and 1.7M (100,000 TDS, highly concentrated brine) of NaCl. Here, we focused on the suspended solids (silica) removal by EC since ICP at high salinity has been extensively studied in another manuscript [6]. As shown in Figure 3A, silica removal at 500mM and 1.7M were similar, with ~100% removal above 75A/m2, which was slightly lower than the required current level of 100A/m2 at 10 mM. The electrical energy consumption (EEC) for 1471 complete silica removal was 5–10mWh/L (Figure 3B). The EEC at 10mM was estimated to be 55mWh/L based on previous experiments. The lower EEC at high salinity is largely due to the lower resistance in the solution. The high concentration of electrolyte could have also enhanced the coagulation process. Additionally, our system was used to remove bacteria (E. coli), which are prevalent in river water in many developing countries [7],[8]. The result in Figure 3C shows effective removal of these bacteria, even at a very high concentration. This shows the flexible applicability of EC to different types of pollutants. Figure 3: (A) & (B)Electrocoagulation performance at high salinity. (A) Silica removal by electro-coagulation at various salinity. (B) Electrical energy consumption per volume of clean water produced. It should be noted that at a low current density, the treated water is not 100% free of silica. (C) EC removal of bacteria (E. Coli). The flowrate was 200µL/min (vavg=1m/min). Salinity was 20 mM NaCl. CONCLUSION This microfluidic EC-ICP platform is, to our knowledge, the first demonstration of electrocoagulation in micro scale. The microfluidic system enables us to visualize the EC process in small scale and facilitates a systematic optimization of various parameters. By combining two different electrochemical processes in the same system, we may eliminate unnecessary voltage drop and achieve both seamless integration and energy efficiency enhancement. We showed that our system can effectively remove colloidal particles, such as suspended solids and bacteria, and salt content. More importantly, our hybrid system is flexible because it can treat a wide range of wastewater with various particles and salt by simply adjusting the operating parameters. From this, we can see that our system can be used in many applications, including river water treatment for domestic water usage in developing countries and further water treatment for irrigation. ACKNOWLEDGEMENTS This work was supported by the Advanced Research Projects Agency-Energy (ARPA-E grant DE‐AR0000294). REFERENCES [1] MM Emamjomeh et al., J Environ Manage. 2009 [2] V Kuokkanen et al., Green Sustain Chem. 2013 [3] R Kwak et al., microTAS, 2013 [4] R Kwak et al., Desalination. 2013 [5] Standard Methods for the Examination of Water and Wastewater, Volume 2, 1915 [6] B Kim et al., microTAS, 2014 [7] G Harris et al., The New York Times. Published March 12, 2013 [8] Pollution C, Board C. Water Quality Monitoring in India – Achievements and Constraints, 2005. CONTACT * Jongyoon Han; phone: +1-617-253-2290; [email protected] 1472 .
Details
-
File Typepdf
-
Upload Time-
-
Content LanguagesEnglish
-
Upload UserAnonymous/Not logged-in
-
File Pages3 Page
-
File Size-