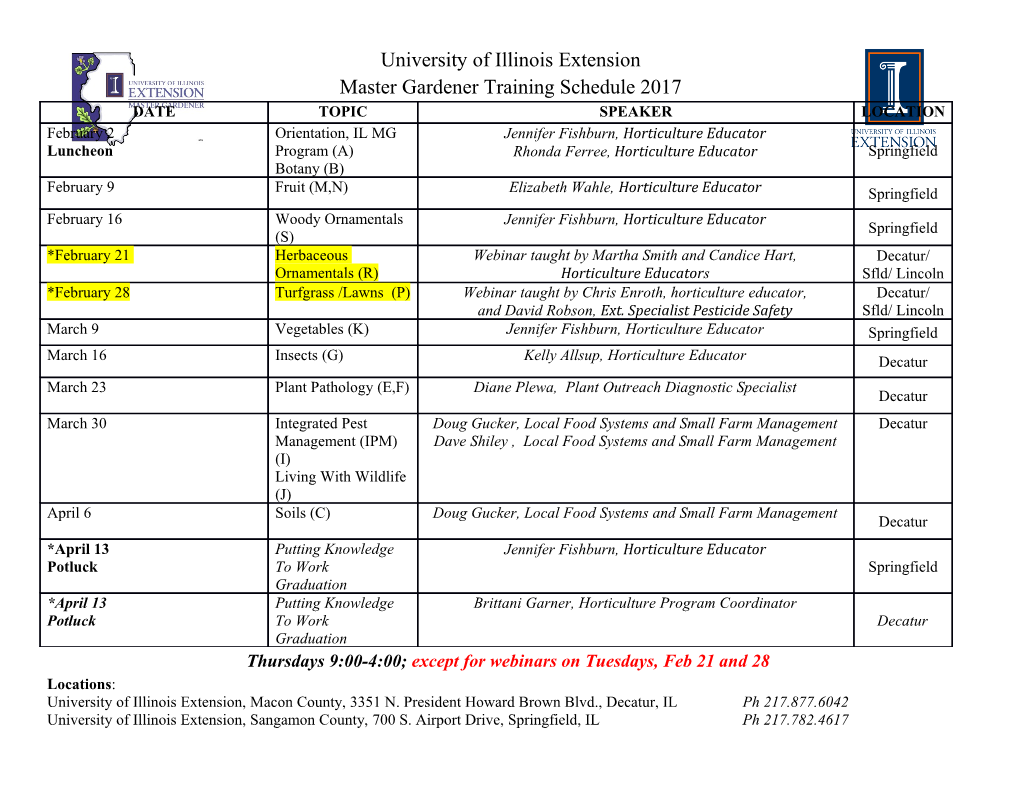
Mapping a Neural Circuit: A Complete Input-Output Diagram in the Primate Retina Greg D. Field1*, Jeffrey L. Gauthier1*, Alexander Sher2*, Martin Greschner1, Timothy Machado1, Lauren H. Jepson1, Jonathon Shlens1, Deborah E. Gunning3, Keith Mathieson3, Wladyslaw Dabrowski4, Liam Paninski5, Alan M. Litke2, and E.J. Chichilnisky1 1 Systems Neurobiology Laboratory, Salk Institute for Biological Studies, La Jolla, CA 2 Santa Cruz Institute for Particle Physics, University of California, Santa Cruz, CA 3 Department of Physics and Astronomy, University of Glasgow, Glasgow, UK 4 Faculty of Physics and Applied Computer Science, AGH University of Science and Technology, 23 30-059, Krakow, Poland 5 Department of Statistics and Center for Theoretical Neuroscience, Columbia University, New York, NY * These authors contributed equally. To understand a neural circuit requires knowing the pattern of connectivity between its inputs and outputs. For example, the role of the retina in color vision depends on the pattern of connectivity between the lattice of (L)ong, (M)iddle and (S)hort-wavelength sensitive cones and multiple types of retinal ganglion cells, each of which samples the visual field uniformly. In the vertebrate nervous system, this kind of comprehensive circuit information has generally been out of reach. Here we report the first measurements detailing functional connectivity between the input and output layers of the primate retina, and we use this information to probe the neural circuitry for color vision. We employed a unique multi-electrode technology to record simultaneously from complete populations of the ganglion cell types that mediate high-resolution vision in primates (midget, parasol, small bistratified). We then used fine-grained visual stimulation to separately identify the location and spectral type of each cone photoreceptor providing input to each ganglion cell. The populations of ON and OFF midget and parasol cells each sampled essentially the complete population of L and M cones. However, only OFF midget cells frequently received at least one strong functional input from an S cone. Statistical analysis revealed a non-random tendency toward pure sampling of either L or M cones in the receptive field centers of midget cells, while inputs to the receptive field surround were apparently random. This purity could not be explained by clumping in the cone mosaic, implying that developmental or adaptive mechanisms enhance red-green color signals sent to the brain. Color vision requires neural circuitry to compare signals from spectrally distinct cone types. For example, the signature of primate color vision – red-green and blue-yellow color opponency – implies that neural circuits pit signals from different cone types against one another. However, the pattern of connectivity between the three cone types and various retinal ganglion cell (RGC) types, which determines how color signals are 1 transmitted in parallel pathways to the brain, remains incompletely understood 1-9. To probe the circuitry for color vision more fully, we measured the pattern of connectivity between the full lattice of cone photoreceptors and complete populations of RGCs of several types in primate retina. We recorded from hundreds of RGCs simultaneously in the peripheral macaque monkey retina using a unique large-scale electrophysiological recording system 10,11. The light response properties of each cell were identified by computing the spike- triggered average (STA) stimulus obtained during presentation of spatio-temporal white noise (see Supplementary Methods). From the STA, several features of the light response were identified, including the spatial receptive field (RF) and the time course of the light response. Clustering of all recorded cells based on these properties was used to identify functionally distinct RGC classes (Fig. 1a, center). The RFs of each cell class formed a regular mosaic uniformly covering the region of retina recorded 11,12. This mosaic organization indicated that each functionally defined cell class corresponded to a single RGC type, because each RGC type uniformly tiles the retinal surface with its dendritic arbors 13. The density of cells in each mosaic was used to identify the ON and OFF midget, ON and OFF parasol, and small bistratified cell types, which collectively account for ~60-70% of RGCs 14. In many cases, the RF mosaics exhibited few or no gaps over a substantial region, indicating that essentially every cell of these types had been recorded. However, traditional RF maps such as these obscure the fact that each RF is composed of the inputs of discrete photoreceptors. To resolve them, RFs were mapped again using white noise stimuli roughly 10-fold finer (pixels 5x5 µm on a side). Measured at this spatial scale, RFs did not conform to the smooth Gaussian approximation used in Fig. 1a (center) and in many previous studies 14. Instead, each RF was composed of multiple punctate islands of light sensitivity, separated by regions of no light sensitivity (Fig. 1a, lower images). The separation was roughly equal to the spacing of cone photoreceptors in the recorded region of retina, consistent with the idea that each island reflected the contribution of a single cone. To test this hypothesis, the locations of the islands were compared to the locations of cone outer segments labeled with peanut agglutinin after the recording; a close alignment was observed (Fig. 1b, see Supplementary Methods). The spectral type of each cone -- (L)ong, (M)iddle, or (S)hort wavelength sensitive -- was identified using the relative magnitudes of the three display primaries in the STA at its spatial location (Fig. 2a). !These values, accumulated across all cones identified in a single recording, formed three distinct clusters (Fig. 2b). !The clusters were closely aligned with predictions based on the spectral sensitivity of the macaque L, M and S cones. !S cones were easily identifiable, L and M cones were somewhat less so because of their overlapping spectral sensitivities (Fig. 2b,c). The full cone mosaic was visualized by pooling cone locations and types across all recorded RGCs (Fig. 2d-e). !This was accomplished by fitting the entire collection of cones to the RF data from all RGCs, simultaneously, using a model in which each RF is a sum of Gaussian functions of fixed size, each centered on the location of a cone (see Supplementary Methods). This fit permitted representation of all identified cones in a 2 single diagram, revealing locally nearly complete cone mosaics (Fig. 2d), and mosaics of varying completeness over larger areas (Fig. 2e). !The relative frequencies of L, M and S cones were in an approximate ratio of 8:4:1 (average) in regions with nearly complete cone mosaics, consistent with previous measurements 14,15. The functional connectivity between each RGC and the cones within its RF was summarized by assigning a weight to each cone, representing the strength of its input. ! The weights were obtained from the fitted model described above. !This approach permitted a well-constrained estimation of the input strength of weak cones, including those in the RF surround (Fig. 2g), because cone locations were robustly identified using data from multiple cells. !The cone inputs to each RGC were summarized using a collection of radiating lines connecting to the cones, with the thickness of each line proportional to the weight, and its shade representing the sign of the input (Fig. 2h). ! This representation permitted simultaneous visualization of a complete mosaic of RGCs and the complete mosaic of the cones they sampled (Fig. 3), i.e. a full functional wiring diagram for the retinal circuits mediating high resolution vision. !A total of 1,961 RGCs connecting to a total of 17,380 cones in 7 preparations were examined. The complete connectivity diagrams provide a powerful new tool for examining specificity of L, M and S cone inputs to different types of RGCs. This specificity has been a source of controversy, in part because previous studies inferred cone inputs indirectly by manipulating the spectral composition of visual stimuli 1-8 (see Supplementary Discussion). The high resolution connectivity maps (Fig. 3) reveal individual L, M and S cone inputs to each RGC resolved on the basis of their spatial location, solving two major open problems about color circuitry in the retina. Previous studies provide conflicting accounts of S cone inputs to midget and parasol RGCs 1,4,6,16 (see Supplementary Discussion). In the present data OFF midget cells frequently received at least one strong functional input from an S cone, whereas ON midget, ON parasol and OFF parasol cells did so much less frequently (e.g. see Fig. 3). At the same time, all four RGC types sampled essentially the entire mosaic of L and M cones. A focused example is given in Fig. 4a-c, which shows the connectivity diagram for an ON midget cell and two OFF midget cells sampling the same region; the S cones were sampled by the OFF midget cells but not the ON midget cell. On average, S cones were strongly sampled by OFF midget cells about five-fold more frequently than by ON midget and parasol cells (Fig. 4d; see Supplementary Methods). Additional analysis showed that ON midget cells displayed a tendency to sample weakly from S cones (see Supplementary Methods). The sampling of S cones by OFF midget cells confirms a prediction from anatomical work 16: OFF midget bipolar cells contact S cones in the central primate retina, therefore, OFF midget cells should receive S cone input. The absence of S cone input to parasol cells also confirms recent findings 6. An important question for future work is whether the S cone signals carried by OFF midget cells contribute to blue-yellow and red-green opponent color vision. The specificity of L and M cone inputs to peripheral midget cells, which is thought to underlie red-green opponent color vision, has been debated extensively (see Supplementary Discussion).
Details
-
File Typepdf
-
Upload Time-
-
Content LanguagesEnglish
-
Upload UserAnonymous/Not logged-in
-
File Pages18 Page
-
File Size-