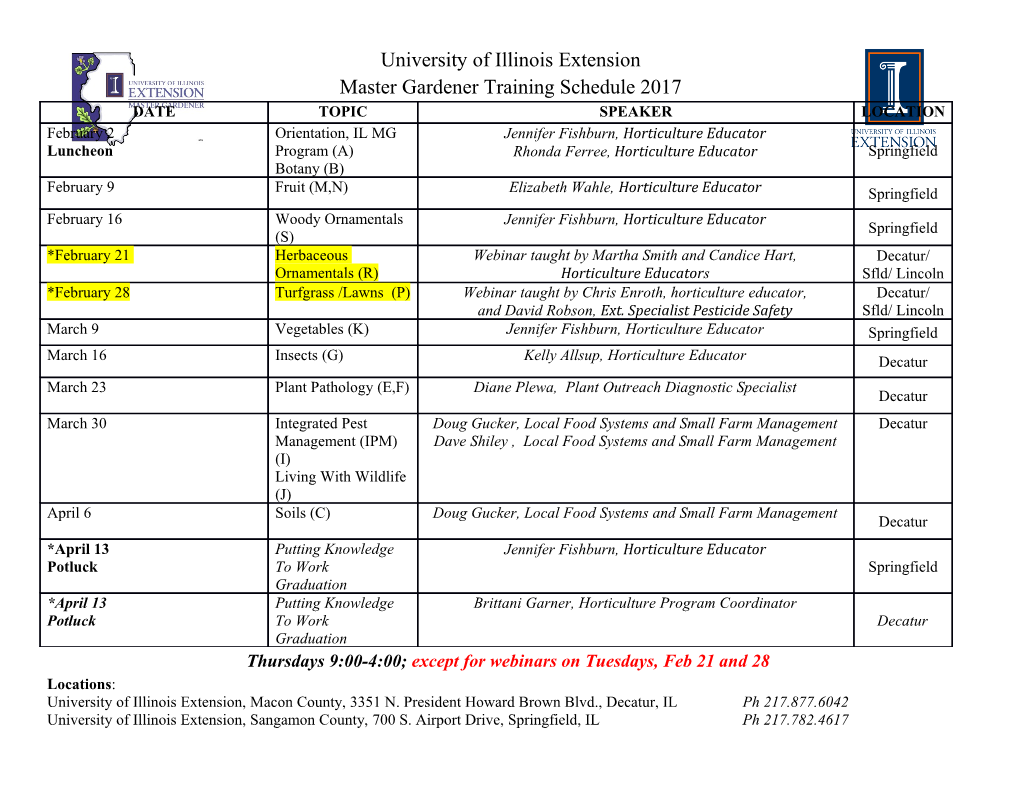
Science Case for the Giant Radio Antenna Neutrino Detector Kumiko Kotera — Institut d’Astrophysique de Paris, UMR 7095 - CNRS, Université Pierre & Marie Curie, 98 bis boulevard Arago, 75014, Paris, France ; email: [email protected] “The title [of this book] is more of an expression of hope than a de- scription of the book’s contents [...]. As new ideas (theoretical and experimental) are explored, the observational horizon of neutrino as- trophysics may grow and the successor to this book may take on a different character, perhaps in a time as short as one or two decades.” John N. Bahcall, Neutrino Astrophysics, Cambridge University Press, 1989 igh-energy (> 1015 eV) neutrino as- progress in the fields of high-energy astro- tronomy will probe the working of physics and astroparticle physics. Addi- Hthe most violent phenomena in the tionally, they should contribute to unveil Universe. When most of the information fundamental neutrino properties. we have on the Universe stems from the observation of light, one essential strategy to undertake is to diversify our messengers. 1 Why neutrinos? The most energetic, mysterious and hardly understood astrophysical sources or events Neutrinos are unique messengers that let (fast-rotating neutron stars, supernova ex- us see deeper in objects, further in dis- plosions and remnants, gamma-ray bursts, tance, and pinpoint the exact location of outflows and flares from active galactic nu- their sources. clei, ...) are expected to be producers Neutrinos can escape much denser as- of high-energy non-thermal hadronic emis- trophysical environments than light, hence sion. Hence they should produce copious they enable us to explore processes in the amounts of high-energy neutrinos. These deepest layers of objects that are invisible signatures will be central to any future to traditional and gamma-ray astronomy. This can be illustrated through the exam- Kumiko Kotera • GRAND Science Case page 1 of 13 ple of MeV neutrinos detected from the downwards, or by using the Earth and/or Sun, which made possible the direct study its atmosphere as a target, and observe of the nuclear interactions occurring at its the produced up-going secondary showers very core. The detection of the MeV neu- (see, e.g., [1]). trinos emitted by the explosion of the lo- cal supernova 1987A also provided a direct From the astrophysical point of view, it observation of the core-collapse mechanism should be pointed out that neutrinos with of massive stars. In both cases, stringent energies much greater than GeV can only constraints were also derived on fundamen- be produced by very high-energy primary tal neutrino properties (masses, oscillation, hadronic cosmic rays, via neutron decay etc.). or by interaction with radiation or mat- Neutrinos travel in geodesics unaffected ter. Note that neutrinos provide definite by magnetic fields. Unlike cosmic-rays evidence for hadronic acceleration, while which are charged, they do not undergo de- gamma-ray signatures are controversial, as flections that induce a shift from the source they can also be generated through leptonic position in the sky as well as time delays1. channels. Neutrinos can thus be spotted in spatial The main goal of the construction of high and temporal coincidence with any type of energy (> PeV) neutrino telescopes is thus source (steady or transient). to explore the signatures of hadronic ac- Cosmic rays and photons are also both celeration in the most powerful phenom- absorbed by the cosmic backgrounds while ena in the Universe. As detailed in the propagating through cosmological scales in following sections, ultrahigh-energy cosmic the Universe. Neutrinos on the contrary ray (UHECR) observations tell us that the are not affected by such backgrounds and highest energy particles are most likely to can easily probe cosmological distances. be produced in extra-galactic sources, and These characteristics could help identify the existence of PeV to EeV (= 1015−18 eV) the origins of high-energy cosmic rays, that neutrinos is guaranteed. By going to high have remained a mystery for over a century. energies, we thus naturally move to neu- trino astronomy at cosmological scales. 2 Why high-energy neutrinos? 3 Neutrinos from ultrahigh The small interaction cross-section of energy cosmic rays: neutrinos makes it difficult to detect theoretical predictions them on the Earth. A first experimental argument for going to high energies, is that The cosmic-ray spectrum extends to en- the interaction probability for neutrinos ergies above 1020 eV. The highest energy roughly scales with energy. Above about cosmic rays are likely to originate in ex- PeV energies, the Earth becomes opaque tragalactic sources, given the (limited) to neutrinos. It is then possible to detect strength of Galactic magnetic fields and them either by looking at those coming the lack of correlations with the Galac- tic plane. Cosmic-ray anisotropy measure- 1a 1-degree deflection over 100 Mpc leads to 104 yrs of time delay compared to a rectilinear ments with the Auger Observatory and the propagation. Telescope Array indicate that the Galactic- Kumiko Kotera • GRAND Science Case page 2 of 13 extragalactic source transition likely hap- pens around a few EeV [2, 3, 4]. A fraction of the UHECR energy is ex- pected to be converted to high energy neu- trinos through the decay of charged pi- ons produced by interactions with ambient matter and radiation2. These interactions may happen either in the source environ- ment, or during the journey of the primary UHECR from the source to the Earth. The neutrinos produced in the latter scenario are called cosmogenic neutrinos. 3.1 Neutrinos from the source environments Figure 1: Estimates for neutrino fluxes for all flavors produced at source environ- For sources where proton are accelerated ments, for clusters of galaxies [5], and undergo photo-pion interactions with GRBs [6], pulsars [7], AGN [8]. optical depth equal to unity (τpγ = 1), The IceCube detection range and the neutrino luminosity derived from the flux level is indicated in red hashes, observed cosmic-ray luminosity is of order and the Waxman-Bahcall bound in red dotted lines. Overlayed are ex- E2Φ ∼ 3.4 × 10−8 GeV cm−2 sr−1 s−1 ν WB perimental limits (current and pro- [9]. This reference estimate is called the jected). Details are given in caption Waxman-Bahcall bound. For optically thin of Fig. 2. sources, the overall level of neutrinos is ex- pected to be lower, and for optically thick sources, cosmic rays are not accelerated to of the injection spectrum as well as on the the highest energies and neutrinos above phenomenological modeling of the acceler- E ∼ EeV are sharply suppressed [10]. ation ([18, 19, 9, 20, 21, 22, 5, 23, 24, 25, The magnetic field in the source envi- 10, 8, 26], see also [27, 28, 29, 30, 6, 31] for ronment, especially in clusters of galax- GRB-specific studies and [32, 7] for neutron ies, can play an important role by confin- stars). Example of flux estimates are pre- ing the charged UHECRs and thus lead- sented in Fig. 1. Note that these estimates ing to increased interaction probabilities do not assume particularly optimistic pa- [11, 12, 13, 14, 15, 5, 16, 17]. rameters. The normalization of these fluxes highly Some source scenarios can however be depends on assumptions about the opacity tested with increased sensitivities. In par- of the acceleration region and on the shape ticular, the sensitivity of IceCube is already highly constraining fireball-type UHECR 2About 1/2 of pγ photopion interactions (2/3 of acceleration models in GRBs. In such pp hadronic interactions) produce charged pi- models, the amount of neutrino produc- ons, that carry a fraction of the parent cosmic- tion in the internal shock regions where ray energy Eπ/Ep ∼ 0.2 (Eπ/Ep ∼ 0.6 for pp). Each neutrino takes up Eν /Eπ ∼ 0.25 of the ions should be accelerated can be calcu- parent pion. lated consistently, once assumptions are Kumiko Kotera • GRAND Science Case page 3 of 13 made on a finite set of parameters such as UHECR models with large proton max- the baryonic loading, the acceleration effi- imum acceleration energy (Emax > 100 ciency to ultrahigh energies, the jet Lorentz EeV), source evolution corresponding to factor, etc. Studies show that the parame- the star formation history or the GRB rate ter space allowed for these quantities would evolution and pure proton or mixed ‘Galac- be strongly reduced if no neutrinos are ob- tic’ compositions, are shaded in grey in Fig- served from the position of detected GRBs ure 2 and give detectable fluxes in the EeV in the next decade [33, 34, 35, 36, 37]. range with 0.06 − 0.2 neutrino per year at Newly-born pulsar scenarios also predict a IceCube and 2 − 5 neutrino per year for guaranteed level of EeV neutrinos by in- GRAND. This energy range should be also teraction of UHECRs in the surrounding soon explored by projected radio instru- supernova ejecta [32, 7]. A non-detection ments such as the Askaryan Radio Array of neutrinos at EeV energies in the next (ARA, [45]) and ARIANNA [47]. decade would enable us to rule out this po- The sensitivity of GRAND nearly tential candidate source. reaches the lowest predicted limits: either a large number of cosmogenic neutrinos will 3.2 The guaranteed cosmogenic be detected, under standard assumptions neutrinos on sources, or extremely severe constraints will be derived for the most pessimistic sce- The constraints derived on UHECRs (en- narios. ergy, nature, ...) from their detection necessarly implies that their propagation in the intergalactic medium leads to the 4 Why now? — the production of cosmogenic neutrinos and multi-messenger and gamma-rays by interactions on the cosmic time-domain era radiation backgrounds (so-called GZK ef- fect).
Details
-
File Typepdf
-
Upload Time-
-
Content LanguagesEnglish
-
Upload UserAnonymous/Not logged-in
-
File Pages13 Page
-
File Size-