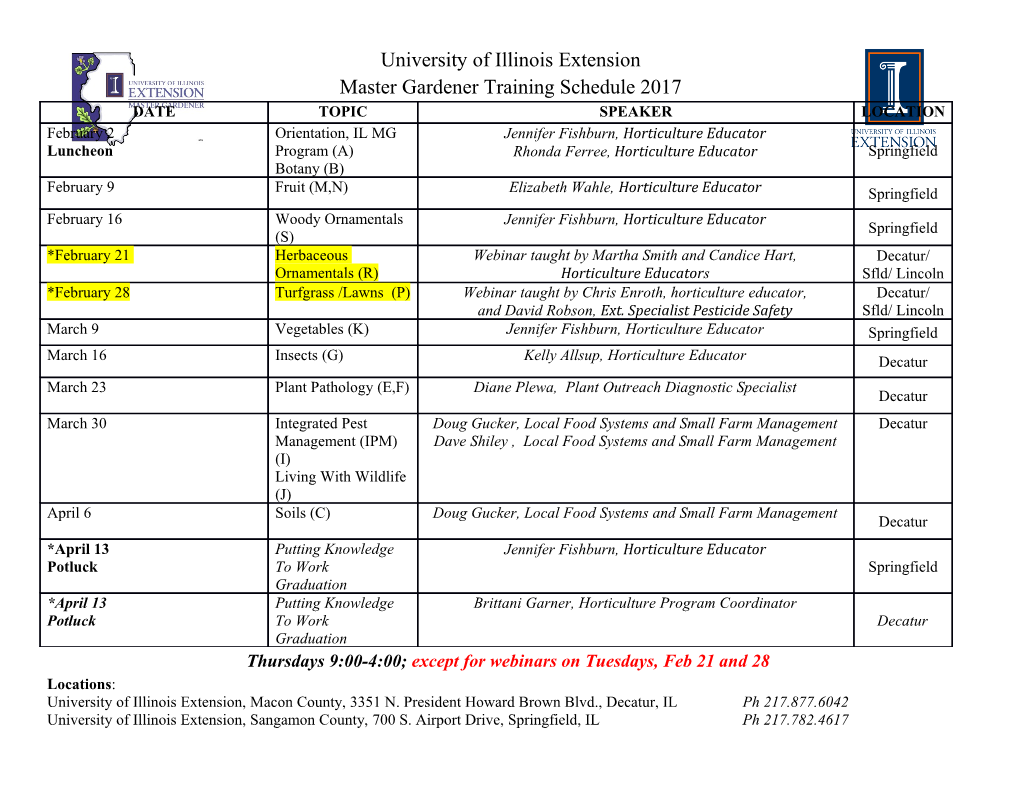
Femtosecond Laser Ablation of Silicon: Nanoparticles, Doping and Photovoltaics A thesis presented by Brian Robert Tull to The School of Engineering and Applied Sciences in partial fulfillment of the requirements for the degree of Doctor of Philosophy in the subject of Applied Physics Harvard University Cambridge, Massachusetts June 2007 c 2007 by Brian Robert Tull All rights reserved. iii Femtosecond Laser Ablation of Silicon: Nanoparticles, Doping and Photovoltaics Eric Mazur Brian R. Tull Abstract In this thesis, we investigate the irradiation of silicon, in a background gas of near atmospheric pressure, with intense femtosecond laser pulses at energy densities exceeding the threshold for ablation (the macroscopic removal of material). We study the resulting structure and properties of the material ejected in the ablation plume as well as the laser irradiated surface itself. The material collected from the ablation plume is a mixture of single crystal silicon nanoparticles and a highly porous network of amorphous silicon. The crystalline nanoparti- cles form by nucleation and growth; the amorphous material has smaller features and forms at a higher cooling rate than the crystalline particles. The size distribution of the crys- talline particles suggests that particle formation after ablation is fundamentally different in a background gas than in vacuum. We also observe interesting structures of coagulated particles such as straight lines and bridges. The laser irradiated surface exhibits enhanced visible and infrared absorption of light when laser ablation is performed in the presence of certain elements|either in the background gas or in a film on the silicon surface. To determine the origin of this enhanced absorption, we perform a comprehensive annealing study of silicon samples irradiated in the presence of three different elements (sulfur, selenium and tellurium). Our results support that the enhanced infrared absorption is caused by a high concentration of dopants dissolved in the lattice. Thermal annealing reduces the infrared absorptance of each doped sample at the same rate that dopants diffuse from within the polycrystalline grains in the laser irradiated surface layer to the grain boundaries. iv Lastly, we measure the photovoltaic properties of the laser irradiated silicon as a function of several parameters: annealing temperature, laser fluence, background gas, surface morphology and chemical etching. We explore the concept of using thin silicon films as the irradiation substrate and successfully enhance the visible and infrared absorp- tion of films ≤ 2 µm thick. Our results suggest that the incorporation of a femtosecond laser modified region into a thin film silicon device could greatly enhance its photovoltaic efficiency. Table of Contents Abstract iii Table of Contents v List of Figures viii List of Tables xi Acknowledgements xii Citations to Published Work xv 1 Introduction 1 1.1 Femtosecond laser irradiation of silicon . .2 1.1.1 Absorption of the pulse . .2 1.1.2 Melting and ablation: thermal regime . .6 1.1.3 Melting and ablation: non-thermal regime . .6 1.1.4 Resolidification of the surface . .7 1.1.5 Comparison to this thesis . .8 1.2 Organization of the dissertation . .9 2 Background 11 2.1 Previous work on femtosecond laser irradiation of silicon in a background gas 12 2.2 Experimental methods: femtosecond laser system . 20 2.2.1 Creating the femtosecond laser pulses . 20 2.2.2 Amplifying the femtosecond laser pulses . 22 2.2.3 Controlling the injection and ejection of the seed pulse . 23 2.2.4 The amplified femtosecond laser pulses . 25 2.2.5 Aligning the amplifier cavity . 25 2.3 Experimental methods: irradiation process and vacuum chamber . 27 3 Formation of silicon nanoparticles by femtosecond laser ablation in a back- ground gas 29 3.1 Chapter abstract . 29 v Table of Contents vi 3.2 Introduction . 30 3.3 Experimental setup . 31 3.4 Results . 32 3.5 Discussion . 39 3.6 Conclusion . 43 4 Mechanism of broadband infrared absorption in chalcogen-doped silicon 45 4.1 Chapter abstract . 45 4.2 Introduction . 46 4.3 Experimental setup . 48 4.4 Results . 50 4.5 Discussion . 53 4.5.1 Diffusion theory . 57 4.5.2 Comparison to diffusion theory . 59 4.5.3 Diffusion theory using a lognormal distribution of grains . 62 4.5.4 Diffusion theory with grain growth . 64 4.6 Conclusion . 70 4.7 Supplemental information . 70 4.7.1 Using an evaporated film as the dopant source . 70 4.7.2 Diffusion coefficients for sulfur, selenium and tellurium in silicon . 74 4.7.3 Absorptance spectra of chalcogen-doped silicon after annealing . 75 4.7.4 Alternate graphs of optical data versus diffusion length . 75 4.7.5 Absorptance spectra of samples irradiated in N2 after annealing . 78 5 The photovoltaic potential of femtosecond laser irradiated silicon 79 5.1 Photovoltaic research . 79 5.2 Laser irradiated silicon solar cells . 82 5.2.1 Thin film silicon . 85 5.2.2 Combining femtosecond laser irradiation and thin film silicon . 86 5.3 The electrical characteristics of a solar cell . 88 5.4 Photovoltaic experimental results and discussion . 92 5.4.1 Measuring current-voltage (I-V ) curves . 92 5.4.2 The effect of annealing on the photovoltaic properties of sulfur-doped femtosecond laser irradiated silicon . 94 5.4.3 The effect of HF acid etching on photovoltaic results . 102 5.4.4 The effect of H2 gas and resulting surface morphology on photovoltaic results . 103 5.4.5 Creating a lower roughness laser-irradiated surface . 109 5.4.6 Irradiating thin film silicon . 115 5.5 Summary discussion . 120 6 Summary and future directions 123 Table of Contents vii A Operation of a solar cell 126 A.1 The photovoltaic cell in an electrical circuit . 126 A.2 Satisfying the two conditions required for a photovoltaic response . 130 A.3 Creating the internal electric field . 132 A.4 The I-V characteristics of the p-n junction . 137 A.5 The ideal material . 145 References 147 List of Figures 1.1 Direct and indirect electron transitions in the electronic band diagram of silicon3 1.2 Absorption coefficient and absorption length of silicon as a function of wave- length . .4 2.1 Images of femtosecond laser irradiated silicon during irradiation and after . 12 2.2 SEM images of femtosecond laser irradiated silicon . 13 2.3 Absorptance spectra of silicon irradiated in different environments . 14 2.4 Cross-sectional TEM analysis of femtosecond laser irradiated silicon . 14 2.5 The effect of annealing on femtosecond laser irradiated silicon . 16 2.6 The effect of annealing on the electrical properties of femtosecond laser irra- diated silicon . 17 2.7 Light detection and photovoltaic response of femtosecond laser irradiated silicon . 18 2.8 Apsorptance spectra of chalcogen-doped femtosecond laser irradiated silicon using a powder source . 19 2.9 Layout of amplifier cavity . 23 2.10 Schematic diagram of the irradiation process and vacuum chamber . 27 3.1 Experimental setup for collecting silicon nanoparticles. 32 3.2 TEM bright field image of particles formed in the ablation plume. 33 3.3 Selected area diffraction of particles formed in the ablation plume. 34 3.4 Analysis of diffraction pattern indicates diamond cubic. 35 3.5 Diffraction of single nanoparticles shows they are single crystals . 36 3.6 TEM bright field and dark field imaging of particles formed in the ablation plume. 36 3.7 TEM image showing that particles aggregate in straight lines. 38 3.8 TEM image showing that particles form bridges. 39 3.9 Size distribution of crystalline nanoparticles formed in the ablation plume. 40 4.1 Absorptance spectra of chalcogen-doped silicon before and after annealing . 51 4.2 Average absorptance from 1250{2500 nm of chalcogen-doped silicon versus annealing temperature at T ≤ 975 K . 51 viii List of Figures ix 4.3 Average absorptance from 1250{2500 nm of chalcogen-doped silicon versus annealing temperature at T ≥ 875 K . 52 4.4 Normalized absorptance of chalcogen-doped silicon versus diffusion length of the respective dopant . 56 4.5 Fraction of dopant atoms remaining inside a sphere of radius, R0, versus diffusion length. 60 4.6 The effect of a lognormal distribution of grains sizes on the fraction of dopant atoms remaining inside grains. 63 4.7 The effect of adding an offset to the lognormal distribution of grains sizes. 64 4.8 Fraction of dopant atoms remaining inside a sphere of radius, R0, versus diffusion length using an imaginary grain boundary. 67 4.9 Comparison of normalized infrared absorptance for selenium-doped silicon to the theory modified for grain growth . 69 4.10 Evolution of surface morphology when using a film as the dopant source . 72 4.11 The effect of film thickness and laser fluence on optical properties . 73 4.12 Absorptance spectra of chalcogen-doped silicon after annealing . 76 4.13 Transmittance and reflectance versus difussion length . 77 4.14 Normalized transmittance and reflectance versus difussion length . 78 4.15 Absorptance spectra of samples irradiated in N2 gas after annealing . 78 5.1 Solar spectrum of the sun at the earth above the atmosphere . 83 5.2 Schematic cross-section of a femtosecond laser irradiated thin film silicon solar cell . 87 5.3 Illuminated and dark current-voltage curve of a commercial polycrystalline silicon solar cell . 89 5.4 Solar simulator and probe station setup . 93 5.5 Illuminated and dark current-voltage curves of sulfur-doped femtosecond laser irradiated silicon . 95 5.6 Illuminated and dark current-voltage curves of sulfur-doped femtosecond laser irradiated silicon annealed between 575{1075 K . 96 5.7 Illuminated and dark current-voltage curves of sulfur-doped femtosecond laser irradiated silicon annealed between 1075{1208 K . 97 5.8 Isc, Voc, FF and η versus temperature for sulfur-doped samples .
Details
-
File Typepdf
-
Upload Time-
-
Content LanguagesEnglish
-
Upload UserAnonymous/Not logged-in
-
File Pages174 Page
-
File Size-