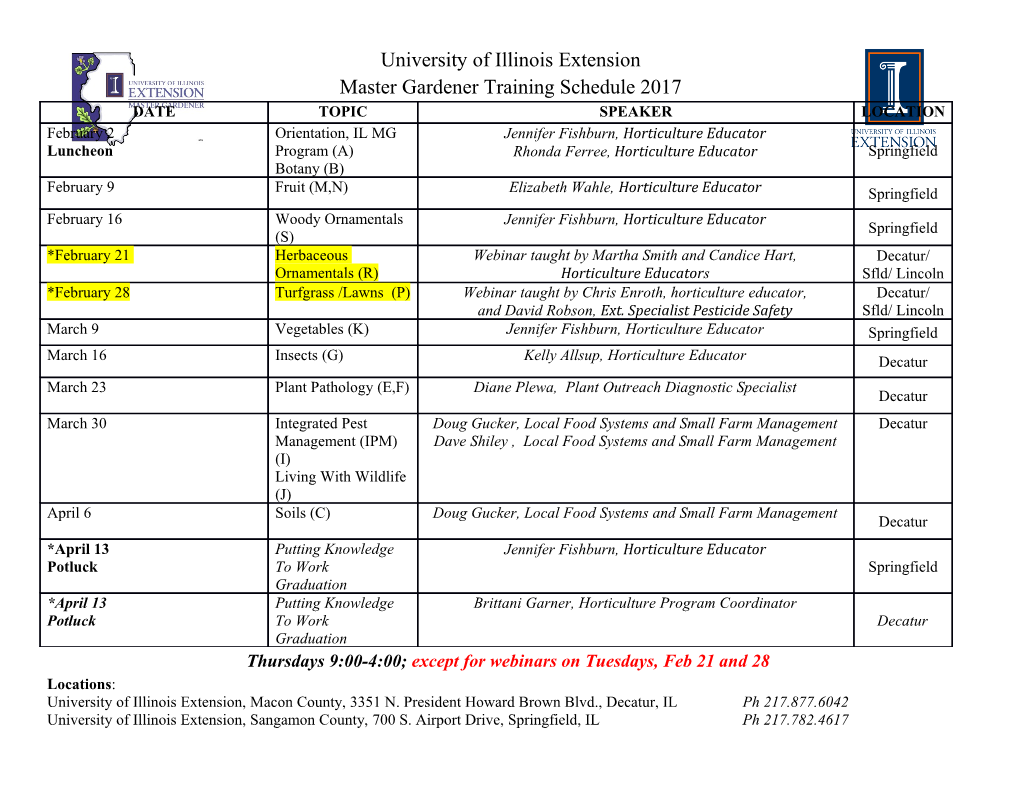
FUNCTIONAL ANALYSIS PIOTR HAJLASZ 1. Banach and Hilbert spaces In what follows K will denote R of C. Definition. A normed space is a pair (X, k · k), where X is a linear space over K and k · k : X → [0, ∞) is a function, called a norm, such that (1) kx + yk ≤ kxk + kyk for all x, y ∈ X; (2) kαxk = |α|kxk for all x ∈ X and α ∈ K; (3) kxk = 0 if and only if x = 0. Since kx − yk ≤ kx − zk + kz − yk for all x, y, z ∈ X, d(x, y) = kx − yk defines a metric in a normed space. In what follows normed paces will always be regarded as metric spaces with respect to the metric d. A normed space is called a Banach space if it is complete with respect to the metric d. Definition. Let X be a linear space over K (=R or C). The inner product (scalar product) is a function h·, ·i : X × X → K such that (1) hx, xi ≥ 0; (2) hx, xi = 0 if and only if x = 0; (3) hαx, yi = αhx, yi; (4) hx1 + x2, yi = hx1, yi + hx2, yi; (5) hx, yi = hy, xi, for all x, x1, x2, y ∈ X and all α ∈ K. As an obvious corollary we obtain hx, y1 + y2i = hx, y1i + hx, y2i, hx, αyi = αhx, yi , Date: February 12, 2009. 1 2 PIOTR HAJLASZ for all x, y1, y2 ∈ X and α ∈ K. For a space with an inner product we define kxk = phx, xi . Lemma 1.1 (Schwarz inequality). If X is a space with an inner product h·, ·i, then |hx, yi| ≤ kxk kyk for all x, y ∈ X. Proof. We can assume that hx, yi 6= 0. For x, y ∈ X and t ∈ R we have 0 ≤ hx + ty, x + tyi = hx, xi + t[hx, yi + hy, xi] + t2hy, yi = hx, xi + 2t re hx, yi + t2hy, yi . We obtained a quadratic function of a variable t which is nonnegative and hence 0 ≥ ∆ = 4(re hx, yi)2 − 4hx, xihy, yi , (re hx, yi)2 ≤ hx, xihy, yi . If |α| = 1, then replacing y by αy we obtain hx, xihy, yi = hx, xihαy, αyi ≥ (re hx, αyi)2 = (re (αhx, yi))2 . In particular for α = hx, yi/|hx, yi| we have !!2 hx, yi hx, xihy, yi ≥ re hx, yi = |hx, yi|2 . |hx, yi| This completes the proof. 2 Corollary 1.2. If X is a space with an inner product h·, ·i, then kxk = phx, yi is a norm. Proof. The properties kαxk = |α|kxk and kxk = 0 if and only if x = 0 are obvious. To prove the last property we need to apply the Schwarz inequality. kx + yk2 = hx + y, x + yi = hx, xi + 2 re hx, yi + hy, yi ≤ kxk2 + 2kxk kyk + kyk2 = (kxk + kyk)2 . Definition. A space with an inner product h·, ·i is called a Hilbert space if it is a Banach space with respect to the norm kxk = phx, xi . Proposition 1.3 (The Polarization Identity). Let h·, ·i be an inner product in X. FUNCTIONAL ANALYSIS 3 (1) If K = R, then 1 hx, yi = (kx + yk2 − kx − yk2) , 4 for all x, y ∈ X. (2) If K = C, then 1 1 hx, yi = kx + yk2 − kx − yk2 − i kix + yk2 − kix − yk2 4 4 for all x, y ∈ X. Proof is left as an easy exercise. Theorem 1.4. Let (X, k · k) be a normed space over K (= R or C). Then there is an inner product h·, ·i such that kxk = phx, xi if and only if the norm satisfies the Parallelogram Law, i.e. kx + yk2 + kx − yk2 = 2kxk2 + 2kyk2 for all x, y ∈ X . The Parallelogram Law has a nice geometric interpretation. Proof. The implication ⇒ follows from a direct computation. To prove the other implication ⇐ we define the inner product using the Polarization Iden- tities and we check that it has all the required properties. We leave the details as an exercise. 2 n 1.1. Examples. 1. C with respect to each of the following norms is a Banach space n X kxk1 = |xi|, i=1 kxk∞ = max |xi|, i=1,2,...,n n !1/p X p kxkp = |xi| , 1 ≤ p < ∞ , i=1 n n where x = (x1, . , xn) ∈ C . The Banach space (C , k · kp) is denoted by p `n. 4 PIOTR HAJLASZ n 2. C with the inner product n X hx, yi = xiyi i=1 is a Hilbert space. Note that the corresponding norm is n !1/2 p X 2 hx, xi = |xi| = kxk2 i=1 2 so `n is a Hilbert space. The metric associated with the norm is v u n uX 2 d(x, y) = t |xi − yi| , i=1 i.e. it is the Euclidean metric. p 3. The spaces `n were defined over K = C, but we can also do the same n n construction for K = R by replacing C by R . The resulting space is also p denoted by `n, but in each situation it will be clear whether we talk about p the real or complex space `n so there is no danger of a confusion. p 4. Consider `2 over K = R. Then the shape of the unit ball {x : kxk ≤ 1} is n 5. A subset of the Euclidean space R is called an ellipsoid if it is the image n n n of the unit ball in R under a nondegenerate linear mapping L : R → R (i.e. det L 6= 0). n n For every ellipsoid E in R there is an inner product in R such that n n E is the unit ball in the associated norm. Indeed, if L : R → R is an isomorphism such that E = L(Bn(0, 1)), then it suffices to define the inner product as hx, yi = (L−1x) · (L−1y) n where · stands for the standard inner product in R . n n More precisely, if L : R → R is non-degenerate, then according to the polar decomposition theorem (P. Lax, Linear Algebra, p. 139) L = RU FUNCTIONAL ANALYSIS 5 where U is unitary and R is positive self-adjoint. The mapping R can be computed explicitly √ LLT = RUU T RT = R2,R = LLT . According to the spectral theorem there is an orthonormal basis v1, . vn in n R (with respect to the standard inner product) such that λ1 0 λ2 R = .. . 0 λn in this basis. That means the mapping L has the following structure. First we rotate (the mapping U) and then we apply R which has a simple geometric meaning of extending the length of vectors v1, . , vn by factors λ1, . , λn. Now if Bn(0, 1) is the unit ball, then U(Bn(0, 1)) is also the unit ball, so n n L(B (0, 1)) = R(B (0, 1)) is an ellipsoid√ with semi-axes λ1v1, . , λnvn of the lengths being eigenvalues of R = LLT . These numbers are called sin- gular values of L. Now the ellipsoid E = L(Bn(0, 1)) is the unit ball for the inner product n n n X X X aibi a v , b v = . i i i i λ2 i=1 i=1 i=1 i We will see later (Corollary 5.12) any real inner product space space H 2 n of dimension n is isometrically isomorphic to `n, i.e. R with the standard 2 inner product, so if L : `n → H is this isometric isomorphism, the unit ball in H is L(Bn(0, 1)), so it is an ellipsoid. Thus we proved. n Theorem 1.5. A convex set in R is a unit ball for a norm associated with an inner product if and only if it is an ellipsoid. ∞ ∞ 6. ` , the space of all bounded (complex, real) sequences x = (an)n=1 with the norm kxk∞ = sup |xn| n is a Banach space. This is very easy to check. 6 PIOTR HAJLASZ 7. c1, the space of all (complex, real) convergent sequences with the norm k · k∞ is a Banach space. 8. c0, the space of all (complex, real) sequences that converge to zero with the norm k · k∞ is a Banach space. ∞ 9. Note that c0 ⊂ c ⊂ ` and both c0 and c are closed linear subspaces of `∞ with respect to the metric generated by the norm. ∞ Exercise. Prove that ` , c and c0 are Banach spaces. ∞ Exercise. Prove that the spaces c and c0 are separable, while ` is not. p ∞ 10. ` , 1 ≤ p < ∞ is the space of all (complex, real) sequences x = (xn)n=1 such that ∞ !1/p X p kxkp = |xn| . n=1 It follows from the Minkowski inequality for sequences that k · kp is a norm and that `p is a linear space. We will prove now that `p is a Banach space, n ∞ p i.e. that it is complete. Let xn = (ai )i=1 be a Cauchy sequence in ` , i.e. for every ε > 0 there is N such that for all n, m > N ∞ !1/p X n m p kxn − xmkp = |ai − ai | < ε . i=1 n ∞ Hence for each i the sequence (ai )n=1 is a Cauchy sequence in K (= C or n R). Let ai = limn→∞ ai . Fix an integer k. Then for n, m > N we have k X n m p p |ai − ai | < ε i=1 and passing to the limit as m → ∞ yields k X n p p |ai − ai| ≤ ε . i=1 Now taking the limit as k → ∞ we obtain ∞ X n p p |ai − ai| ≤ ε , i=1 i.e.
Details
-
File Typepdf
-
Upload Time-
-
Content LanguagesEnglish
-
Upload UserAnonymous/Not logged-in
-
File Pages173 Page
-
File Size-