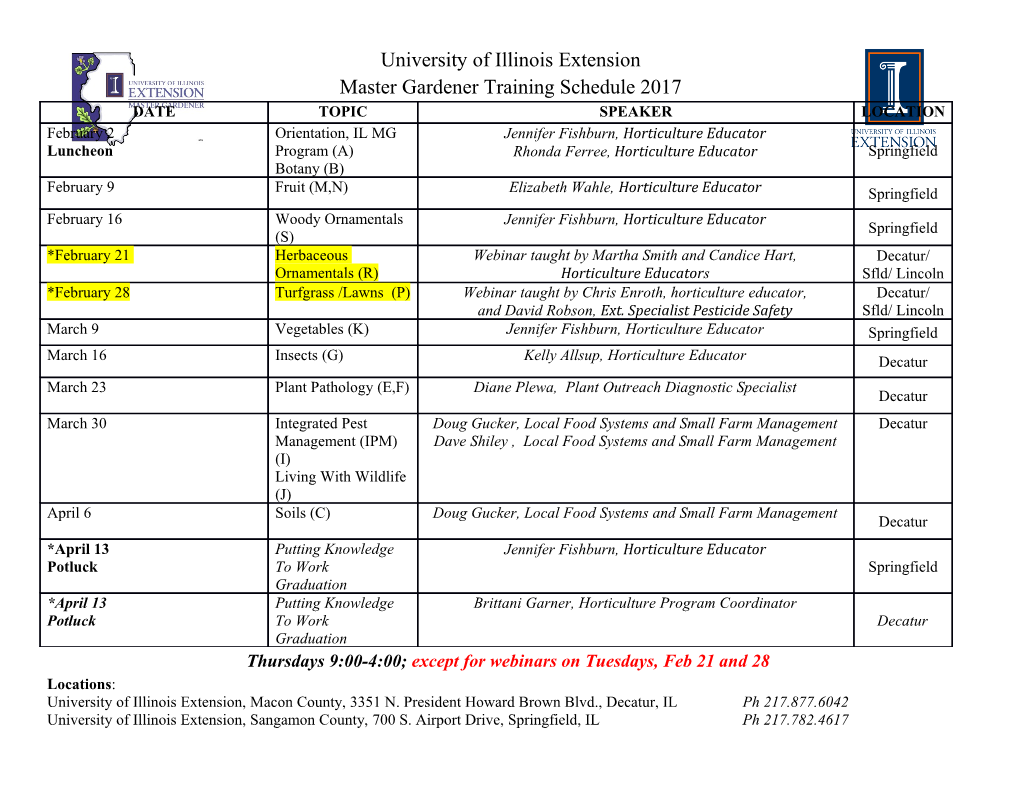
This is a repository copy of Nucleation and growth of todorokite from birnessite: Implications for trace-metal cycling in marine sediments. White Rose Research Online URL for this paper: http://eprints.whiterose.ac.uk/81100/ Version: Accepted Version Article: Atkins, AL, Shaw, S and Peacock, CL (2014) Nucleation and growth of todorokite from birnessite: Implications for trace-metal cycling in marine sediments. Geochimica et Cosmochimica Acta, 144. 109 - 125. ISSN 0016-7037 https://doi.org/10.1016/j.gca.2014.08.014 Reuse Unless indicated otherwise, fulltext items are protected by copyright with all rights reserved. The copyright exception in section 29 of the Copyright, Designs and Patents Act 1988 allows the making of a single copy solely for the purpose of non-commercial research or private study within the limits of fair dealing. The publisher or other rights-holder may allow further reproduction and re-use of this version - refer to the White Rose Research Online record for this item. Where records identify the publisher as the copyright holder, users can verify any specific terms of use on the publisher’s website. Takedown If you consider content in White Rose Research Online to be in breach of UK law, please notify us by emailing [email protected] including the URL of the record and the reason for the withdrawal request. [email protected] https://eprints.whiterose.ac.uk/ 1 Nucleation and growth of todorokite from birnessite: Implications for trace- 2 metal cycling in marine sediments 3 4 Amy L. Atkins1, Samuel Shaw2 and Caroline L. Peacock1* 5 6 1University of Leeds, School of Earth and Environment, Leeds, LS2 9JT, UK. 7 2University of Manchester, School of Earth, Atmospheric and Environmental 8 Sciences, Manchester, M13 9PL, UK. 9 10 11 Submitted to Geochimica et Cosmochimica Acta 12 4th April 2014 13 Revised version submitted 7th August 2014 14 15 *Corresponding author e-mail: [email protected] 1 16 ABSTRACT 17 18 The phyllomanganate birnessite is the main Mn-bearing phase in oxic marine 19 sediments, and through coupled sorption and redox exerts a strong control on the 20 oceanic concentration of micronutrient trace metals. However, under diagenesis and 21 mild hydrothermal conditions, birnessite undergoes transformation to the 22 tectomanganate todorokite. The mechanistic details of this transformation are 23 important for the speciation and mobility of metals sequestered by birnessite, and are 24 necessary in order to quantify the role of marine sediments in global trace element 25 cycles. Here we transform a synthetic, poorly crystalline, hexagonal birnessite, 26 analogous to marine birnessite, into todorokite under a mild reflux procedure, 27 developed to mimic marine diagenesis and mild hydrothermal conditions. We 28 characterize our birnessite and reflux products as a time series, employing X-ray 29 diffraction (XRD), Fourier transform infrared spectroscopy (FTIR), BET surface area 30 analysis, scanning electron microscopy (SEM), high-resolution transmission electron 31 microscopy (HR-TEM) and extended X-ray absorption fine structure spectroscopy 32 (EXAFS). We provide new insight into the crystallization pathway and mechanism of 33 todorokite formation from birnessite under conditions analogous to those found in 34 marine diagenetic and hydrothermal settings. Specifically we propose a new four- 35 stage process for the transformation of birnessite to todorokite, beginning with 36 todorokite nucleation, then crystal growth from solution to form todorokite primary 37 particles, followed by their self-assembly and oriented growth via oriented attachment 38 to form crystalline todorokite laths, culminating in traditional crystal ripening. We 39 suggest that, contrary to current understanding, trace metals like Ni might retard the 40 transformation of birnessite to todorokite and be released to marine sedimentary pore- 41 waters during this diagenetic process, thus potentially providing a benthic flux of 42 these micronutrients to seawater. 43 44 KEYWORDS: Mn oxide; vernadite; birnessite; buserite; todorokite; oriented 45 attachment; self-assembly; diagenesis; marine sediments. 2 46 1. INTRODUCTION 47 Manganese oxides are ubiquitous in oxic marine sediments and play a 48 fundamental role in the biogeochemical cycling of trace elements in the ocean (e.g., 49 Goldberg, 1954; Cronan, 1976; Burns and Burns, 1979; Post, 1999; Peacock and 50 Sherman, 2007a; Sherman and Peacock, 2010; Spiro et al., 2010). Specifically, in 51 addition to their ubiquity, they have large surface areas and are highly reactive, and so 52 through coupled sorption and redox reactions are able to exert a strong control on the 53 speciation, mobility and bioavailability of trace metals and micronutrients (e.g., Post, 54 1999). 55 In oxic marine sediments the main Mn-bearing phases are birnessite, buserite 56 and todorokite (e.g., Burns and Burns, 1977). These minerals are all mixed 57 Mn(III/IV) oxides, but birnessite and buserite are phyllomanganates (layer-type), 58 consisting of sheets (layers) of edge-sharing MnO6 octahedra, whereas todorokite is a 59 tectomanganate (tunnel-type), consisting of triple chains of edge-sharing MnO6 60 octahedra that share corners to form 3D tunnels. In birnessite and buserite, individual 61 phyllomanganate sheets are stacked and separated by a hydrated interlayer region, 62 which is partially filled with water molecules and a variety of cations to balance 63 negative charge generated within the octahedral layers. Birnessite interlayers contain 64 a single layer of water molecules resulting in an interlayer spacing of ~7 and, for 65 example, Na+ interlayer cations, while buserite interlayers contain a double layer of 66 water molecules resulting in an interlayer spacing of ~10 and, for example, Mg2+ 67 interlayer cations. In ideal todorokite, the tunnels have a square cross-section that 68 measures three octahedra wide each side (Burns et al., 1985; Post and Bish, 1988; 69 Post et al., 2003). In this case the tunnels equate to ~10x10 . In the marine 70 environment, todorokite tunnels are typically occupied by hydrated Mg2+ ions (e.g., 71 Bode et al., 2007), whereas in more rarely occurring terrestrial todorokites, other 72 large cations such as Ba2+ are thought to concentrate in the tunnel positions (e.g., Xu 73 et al., 2010). The 7 and 10 phyllomanganates often display a number of structural 74 and physiochemical variations, most notably possessing either triclinic or hexagonal 75 symmetry, where hexagonal symmetry results from ordered vacancies in the 76 manganese octahedral sites (Drits et al., 1997). In addition, these minerals can also 77 exhibit varying degrees of disorder in the stacking of the phyllomanganate sheets 78 along the c-axis, creating a continuum between turbostratic vernadite that consists of 79 randomly orientated sheets (where the synthetic analogue is δ-MnO2), to c-disordered 3 80 phases that display some degree of sheet stacking, to highly crystalline phases in 81 which sheets are ordered over several hundreds of angstroms (e.g., Villalobos et al., 82 2003). Natural marine birnessite is most commonly identified as a hexagonal, poorly 83 crystalline phase, with either incoherent (vernadite) or semi-coherent (c-disordered 84 birnessite) sheet stacking (e.g., Calvert and Price, 1970; Jauhari, 1987; McKenzie, 85 1989; Peacock and Sherman, 2007b; Grangeon et al., 2008). Furthermore, this suite 86 of phyllomanganates also show variations in the proportion of Mn(III)/Mn(IV) within 87 the layers. In vernadite Mn is predominantly present in the +4 oxidation state (e.g., 88 Villalobos et al., 2003), while c-disordered and more crystalline phases contain a 89 higher proportion of structural Mn(III) (e.g., Villalobos et al., 2003; Livi et al., 2011). 90 In the natural environment Mn(III/IV) oxides are thought to form via the 91 microbial oxidation of Mn(II) (e.g., Brouwers, 2000; Francis et al., 2001; Bargar et 92 al., 2000, 2005; Villalobos et al., 2003; Tebo et al., 2005; Webb et al., 2005a, b; 93 Saratovsky et al., 2006; Spiro et al., 2010). However, although todorokite is often 94 found intimitely associated with vernadite and both 7 and 10 semi-coherently 95 stacked phyllomanganates (here collectively termed poorly crystalline 96 phyllomanganates) in marine ferromanganese precipitates (e.g., Burns and Burns, 97 1978a, b; Siegel and Turner, 1983; Usui and Terashima, 1997; Banerjee et al., 1999; 98 Bode et al., 2007; Peacock and Moon, 2012) it is thought to only form during the 99 transformation of a 10 phyllomanganate under diagenetic and low temperature 100 hydrothermal conditions (e.g., Burns and Burns, 1978b). Accordingly, marine 101 ferromanganese precipitates that have precipitated directly from the water column 102 (hydrogenetic) are typically rich in poorly crystalline phyllomanganates, those that 103 have precipitated at the sediment-seawater interface (diagenetic) contain both poorly 104 crystalline phyllomanganates and todorokite in variable proportions reflecting the 105 influence of sediment pore-waters on individual precipitates, while those that have 106 precipitated below the sediment-seawater interface in close proximity to hydrothermal 107 fluids are generally todorokite rich (e.g., Burns and Burns, 1977). 108 Despite the close association of poorly crystalline phyllomanganates and 109 todorokite in natural marine precipitates, the exact crystallization pathway and 110 formation mechanism of todorokite from these minerals in natural environments is 111
Details
-
File Typepdf
-
Upload Time-
-
Content LanguagesEnglish
-
Upload UserAnonymous/Not logged-in
-
File Pages44 Page
-
File Size-