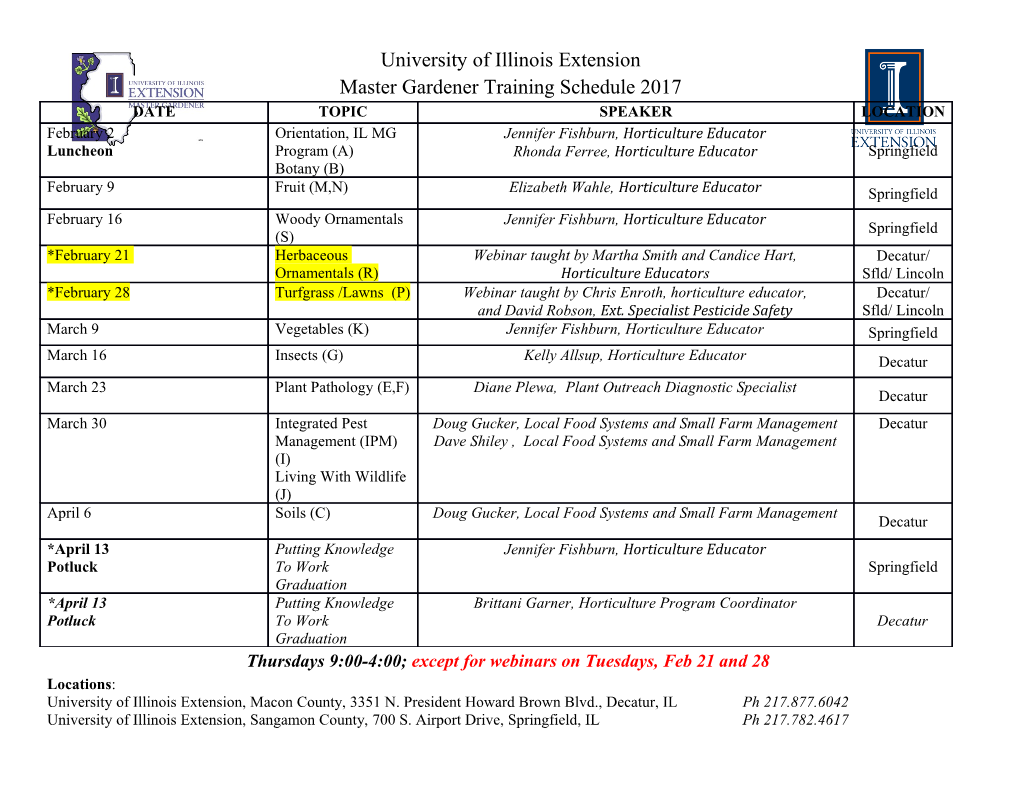
HYPOTHESIS PUBLISHED: 3 APRIL 2017 | VOLUME: 3 | ARTICLE NUMBER: 17041 A mechanism for water splitting and oxygen production in photosynthesis James Barber* Sunlight is absorbed and converted to chemical energy by photosynthetic organisms. At the heart of this process is the most fundamental reaction on Earth, the light-driven splitting of water into its elemental constituents. In this way molecular oxygen is released, maintaining an aerobic atmosphere and creating the ozone layer. The hydrogen that is released is used to convert carbon dioxide into the organic molecules that constitute life and were the origin of fossil fuels. Oxidation of these organic molecules, either by respiration or combustion, leads to the recombination of the stored hydrogen with oxygen, releasing energy and reforming water. This water splitting is achieved by the enzyme photosystem II (PSII). Its appearance at least 3 billion years ago, and linkage through an electron transfer chain to photosystem I, directly led to the emergence of eukaryotic and multicellular organisms. Before this, biological organisms had been dependent on hydrogen/ 2+ electron donors, such as H2S, NH3, organic acids and Fe , that were in limited supply compared with the oceans of liquid water. However, it is likely that water was also used as a hydrogen source before the emergence of PSII, as found today in anaerobic prokaryotic organisms that use carbon monoxide as an energy source to split water. The enzyme that catalyses this reaction is carbon monoxide dehydrogenase (CODH). Similarities between PSII and the iron- and nickel-containing form of this enzyme (Fe-Ni CODH) suggest a possible mechanism for the photosynthetic O–O bond formation. n photosystem II (PSII), the energy of four photons (4 hv) of light details of this final oxidation state are unknown because O–Obond is used by the oxygen-evolving complex (OEC) to drive the formation is very fast. The cycle is powered by the oxidation of a Isplitting of two water molecules to produce dioxygen and four chlorophyll, known as P680, generated by light-driven primary reducing equivalents (equation (1)): charge separation in the reaction centre of PSII coupled to a redox-active tyrosine (YZ), serving as an intermediate electron −4→hn + − + + + 7 2H2O O2 4e 4H (1) carrier between P680 and the Mn cluster (see Fig. 1) . Understanding the molecular mechanism of O–O bond In Fe-Ni CODH, the energy released by the oxidation of a CO formation in PSII during the S-state cycle is one of the most molecule is used to split one water molecule to produce carbon outstanding challenges of bioinorganic chemistry. The importance dioxide and two reducing equivalents (equation (2)): of this cannot be overstated since splitting water into its elemental constituents is thermodynamically and chemically demanding, + −→ + − + + CO H2O CO2 2e 2H (2) being accomplished by the relatively low energy content of four photons of long-wavelength visible light. Over the years, This chemistry is the well-known “water-gas shift reaction” there have been many postulates of the mechanism for O–O bond discovered in 1780 by the Italian physicist Felice Fontana1, and formation in PSII8–12. Here, I present support for a chemical today adopted as a large-scale industrial process to make pure mechanism which is consistent with the structure of the catalytic hydrogen gas from carbon monoxide. This commercial process centre of PSII and which shares striking similarities with the requires shifts in reaction temperature from high (approximately proposed mechanism of water splitting by Fe-Ni CODH13. 400 °C with an iron-chromium oxide catalyst) to low (approximately I, together with my colleagues at Imperial College London14, 225 °C with copper-based catalysts)2. concluded from X-ray diffraction analysis of PSII crystals at 3.5 Å However, in the case of Fe-Ni CODH, the generated reducing resolution, that the oxygen-generating catalytic centre of PSII 2+ “ ” equivalents are produced at ambient temperatures and are used consisted of a Mn3Ca O4 cubane with a fourth dangler Mn to drive the reductive chemistry of the organism rather than attached to the cubane via one of its bridging oxo bonds. Further fi 2+ 15 producing hydrogen gas, usually by electron transport involving re nement of this Mn4Ca O4 model at 1.9 Å by Umena et al. 3,4 fi nearby Fe4-S4 centres . con rmed this geometry but added one additional bridging oxo For PSII, it was elegantly demonstrated that it took four flashes of between the external dangler Mn and the cubane, to make a 2+ 2+ light to obtain the maximum yield of molecular oxygen which was Mn4Ca O5 cluster (see Fig. 2a). Recently, a Mn4Ca O4 cluster fi interpreted as a catalytic cycle consisting of ve main states (S0 to S4), has been synthesized as a pure inorganic molecule with no whereby oxidizing equivalents of about the same potential were protein ligands16 and is essentially identical to that originally sequentially accumulated at the catalytic site with each flash proposed by Ferreira et al.14. As far as it is known, no other 5 (Fig. 1) . The S4 state stores the four oxidizing equivalents needed manganese enzyme has a comparable structure but the catalytic to oxidize two water molecules and in so doing reverts back to the centre of Fe-Ni CODH has remarkably similar geometry (Fig. 2b). 13,17 S0 state. The oxidizing equivalents are stored by four Mn ions in X-ray crystallography at high resolution has shown that Fe-Ni “ ” the catalytic centre leading to four Mn(IV) ions in the S3 state CODH consists of an Fe3NiS4 cubane with a fourth dangler Fe 6 just before the last photochemical step to the S4 state . The precise attached to the cubane via a bridging S of the cubane and an Department of Life Sciences, Imperial College London, Sir Ernst Chain Building, South Kensington Campus, London SW7 2AZ, UK. *e-mail: [email protected] NATURE PLANTS 3, 17041 (2017) | DOI: 10.1038/nplants.2017.41 | www.nature.com/natureplants 1 © 2017 Macmillan Publishers Limited, part of Springer Nature. All rights reserved. HYPOTHESIS NATURE PLANTS O a b c 2 Mn2 Fe3 hν 2H2O Ni Fe2 P680 Mn3 Ca Y S + z 4 1 ms H+ Fe4 Fe1 P680·+ H+ Mn4 Mn1 ·+ Yz S0 S + Figure 2 | Comparison of the Mn Ca2+O cluster of PSII with Fe Ni2+S 3 + Y 4 5 4 5 H z 2+ 30 µs P680 cluster of CODH. a,MnCa O cluster of PSII at 1.9 Å using PDB 3WU2 ·+ + 4 5 Y H 2+ z 350 µs (ref. 15). b,FeNi S cluster of CODH at 1.03 Å using PDB 4UDX (ref. 49). P680·+ 4 5 ·+ hν c, Overlay of the two structures with a RMSD of 0.65 between the metal Yz · Y S P680 + z S2+ 100 µs 1 atoms. Oxo bonds are in red, sulfur bonds in yellow, manganese ions in P680 purple, calcium and nickel ions in green as labelled and iron ions in orange. Y ·+ z Yz hν cluster of PSII derived from X-ray crystallography by myself and P680·+ P680 colleagues was consistent with this proposed mechanism and this was discussed in our paper14. Importantly, the dangler Mn4 was ν h found to be immediately adjacent to the Ca2+ and close to side chains of several key amino acids, including the redox-active Figure 1 | The S-state cycle showing how the absorption of four photons of tyrosine, Y , and therefore we suggested that Mn4 and Ca2+ form hv Z light ( ) by P680 drives the splitting of two water molecules and a ‘catalytic’ surface outside the cubane for binding the two substrate formation of O through a consecutive series of five intermediates (S ,S, 2 0 1 water molecules and their subsequent oxidation. The progression S ,S and S ). Protons (H+) are released during this cycle except for the S 2 3 4 1 through the S-state cycle would lead to deprotonation of these to S transition. Electron donation from the Mn Ca2+ cluster to P680•+ is 2 4 water molecules and the formation in S of a highly acidic electro- aided by the redox-active tyrosine Y . Each step involves a single oxidation 4 Z philic Mn(V)-oxo or Mn(IV)-oxyl radical, and to a nucleophilic of a Mn ion in the cluster, starting at S with 3 Mn(III) + Mn(IV) advancing 2+ 0 hydroxyl on the Ca . The formation of the O–O bond of dioxygen to S with 4 Mn(IV). The exact oxidation state of S is unknown but could 3 4 would result from the nucleophilic attack of hydroxyl on the electro- be 3 Mn(IV) + Mn(V) or 3 Mn(IV) + Mn(IV)-oxyl radical (see below). Also philic oxo of Mn4 with a possible peroxide intermediate. The elec- shown are half-times for the various steps of the cycle. trophilicity of Mn(V)-oxo would be enhanced by the high oxidation level of the cubane (3xMn(IV) plus a net positive charge generated additional S bridge, to make an Fe4NiS5 cluster as shown in Fig. 2b. during the S1 to S2 transition). Therefore, this mechanism of Despite the two catalytic centres being composed of different oxygen atom transfer is very similar to that proposed for Fe-Ni elements, the overlay of their structures, shown in Fig. 2c, is CODH13 and shown diagrammatically as a comparison in Fig. 3. remarkably good with a respectable root-mean-square deviation We also identified seven amino acids as possible ligands to the 14 (RMSD) of 0.65 between the metal atoms. In fact, they are the Mn4Ca cluster , six from the D1 reaction centre protein: only known examples of catalytic centres having heterocubanes D1Asp170, D1Glu189, D1His332, D1Ala344, D1Glu333 and with an additional metal in exo.
Details
-
File Typepdf
-
Upload Time-
-
Content LanguagesEnglish
-
Upload UserAnonymous/Not logged-in
-
File Pages5 Page
-
File Size-