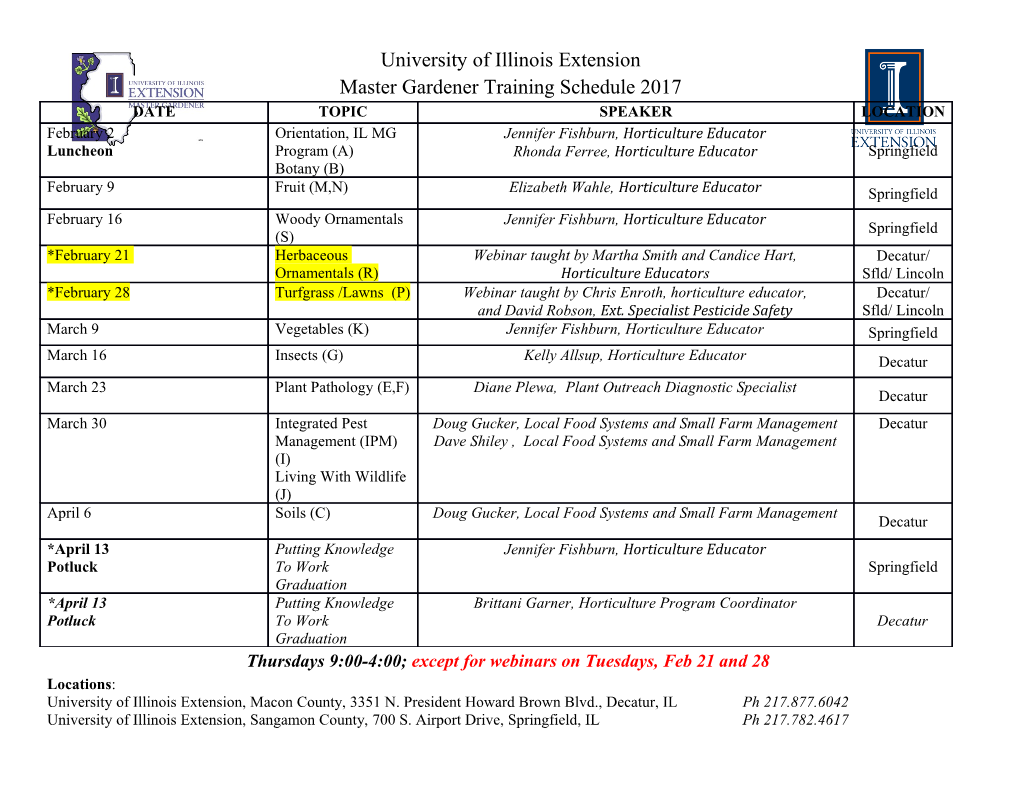
Integrated superconducting detectors on semiconductors for quantum optics applications M. Kaniber,1 F. Flassig,1 G. Reithmaier,1 R. Gross,2,3 and J. J. Finley1,3 1 Walter Schottky Institut and Physik Department, Technische Universität München, Am Coulombwall 4, 85748 Garching, Germany 2 Walther-Meißner-Institut, Bayerische Akademie der Wissenschaften und Physik-Department, Technische Universität München, 85748 Garching, Germany 3 Nanosystems Initiative Munich, Schellingstraße 4, 85748 München, Germany Abstract: Semiconductor quantum photonic circuits can be used to efficiently generate, manipulate, route and exploit non-classical states of light for distributed photon based quantum information technologies. In this article, we review our recent achievements on the growth, nanofabrication and integration of high- quality, superconducting Niobium nitride thin films on optically active, semiconducting GaAs substrates and their patterning to realise highly efficient and ultrafast superconducting detectors on semiconductor nanomaterials containing quantum dots. Our state-of-the-art detectors reach external detection quantum efficiencies up to 20 % for ∼ 4 �� thin films and single photon timing resolutions < 72 ��. We discuss the integration of such detectors into quantum dot loaded, semiconductor ridge waveguides, resulting in the on-chip, time-resolved detection of quantum dot luminescence. Furthermore, a prototype quantum optical circuit is demonstrated that enabled the on-chip generation of resonance fluorescence from an individual InGaAs quantum dot, with a linewidth < 15 ��� displaced by 1 �� from the superconducting detector on the very same semiconductor chip. Thus, all key components required for prototype quantum photonic circuits with sources, optical components and detectors on the same chip are reported. Main text: Semiconductors are ubiquitous in modern opto-electronics and quantum photonic devices and are also expected to play a major role in photonic quantum technologies [1]. In particular, semiconductor quantum dots have been shown to be near ideal sources of non-classical light [2] [3] that can be efficiently routed into nanoscale photonic circuits [4] [5] and even be used to manipulated the quantum state of single photons [4] [6] [7]. Besides the generation and routing of quantum light on a semiconductor chip [3] [8] [9], a further major requirement for realising integrated quantum devices is the on-chip detection of single photons with near unity quantum efficiency [10] and the integration of such detectors into nano-photonic circuits, consisting of waveguides, high-finesse nanocavities and beam splitters, opening the way toward integrated photon mediated quantum information technologies. Superconducting single photon detectors (SSPDs) based on Niobium nitride (NbN) nanowires combine high detection efficiency, low dark count rates, and picosecond timing resolution [11] [12], and can be integrated into prototype optical chips in a straightforward manner [13]. Altogether, the integration of SSPDs into nano-photonic circuits, their high detection efficiencies and ultra-fast response make them promising candidates for numerous quantum information applications [1], such as linear optical quantum computation [14], on-chip photonic quantum gates [15] [16], optical transistors [17] [18] or even quantum repeaters [19] [20]. In this article, we review our recent progress in the growth of high-quality superconducting NbN thin films with critical transition temperatures of �3 > 12 � and their patterning to realise highly efficient (� > 20%) and ultra-fast (< 72 ��) SSPDs on semiconducting GaAs substrates [21]. Furthermore, we demonstrate the integration of such SSPDs in quantum dot loaded photonic waveguides for on-chip time-resolved photoluminescence spectroscopy [22] [23]. Finally, we present a first prototype architecture of a fully integrated photonic nano-circuit, consisting of an individual InGaAs quantum dot, a GaAs/AlGaAs ridge waveguide and a waveguide-integrated SSPD, and demonstrate on-chip resonant fluorescence as a initial step towards on-chip quantum coherent information processing [24] [13]. Niobium nitride thin films were deposited on clean semiconducting gallium arsenide (GaAs) substrates using direct current (DC) reactive magnetron sputtering. This deposition technique has been shown to provide excellent control over the growth temperature �78, nitrogen partial pressure �:; or sputtering rate �:=:. High-quality superconducting NbN thin films have already been realized on various other substrates, for example sapphire [25], MgO [26], or glass [27], using this technique. The ∼ 5×5 ��? GaAs substrates were mounted on a copper sample holder which can be heated up to 850 °�. After FG conditioning, the sputtering chamber is evacuated to a base pressure of �C ≤ 5 ⋅ 10 ���� in order to reduce the partial pressure of residual gases that might become incorporated into the superconducting films as defects and, thus, potentially diminish their superconducting metrics such as critical temperature �K or transition width ��K. After conditioning, carefully measured quantities of process gases, Argon (Ar) and Nitrogen (N2), are introduced into the growth chamber, controlled using mass- flow controllers. The mixture of these gases, i.e. their volume ratio primarily controls the Nb:N stoichiometry and, therefore, influences the resulting crystal structure of the NbN films [28] [21]. After reaching stable conditions with respect to gas flow and pressure, the plasma is started by applying a negative DC voltage of ∼ 350 �, thus, accelerating Ar+ ions towards the sputter target. During this pre- sputtering for ∼ 15 ���, the top-oxide layer of the Nb-target is removed and a total chamber pressure FS of typically �OPO = 4.4 ⋅ 10 ���� is reached. After opening the shutter in front of the GaAs substrates the actual thin film deposition starts, whereby Nb atoms hit the sample surface and subsequently form chemical bonds with N2 and, thus, arrange into crystal grains with a size and crystal structure that depends strongly on the chosen sputtering parameters. Typical film thicknesses investigated are in the range of 5 − 25 ��, determined by atomic force microscopy performed post growth. The superconducting NbN thin films are electrically characterized employing temperature dependent transport measurements using a four-point geometry [29]. A typical result of the film resistance � as a function of temperature � is shown by the blue curve in Figure 1 (a) for a NbN film with thickness �:=: = 26.0 ± 0.5 ��. We observe a gradual decrease of � with decreasing � with a drop to a value close to 0� for � ≤ 11.8 �, reflecting the normal to superconducting phase transition. From such measurements, we deduce the two main figures of merit of the NbN films, namely the critical temperature �K and transition width ��K. Both quantities are extracted from the film resistivity measurements according the analysis in Ref. [30]. Based on the normalized numerical derivative ��/�� as shown by the red curve in Figure 1(a), we define the temperature �"#$%&' at which the film remains just above the transition but still in its normal conducting state, and the �)*+,$ where the film becomes superconducting. We define + ( & + 0 + (' 2 (--1 ) ($ ) 30 " & ' * ) ' ) ! $ ' -' /.' .' $, "#$% & Figure 1 (a) Measured film resistance � and derivative ��/�� as a function of temperature � for a NbN thin film with thickness �CDC = 26.0 ± 0.5 �� in red and blue, respectively. (b) Measured current � as a function of applied bias voltage �DY&) for a NbN SSPD with thickness �CDC = 20.0 ± 0.5 �� as a temperature � = 4 �. Inset: Schematic of electrical circuit used for SSPD electro-optical characterisation. now the average normal state resistivity � as the arithmetic mean of the resistance between �"#$%&' and �)*+,$ + 2 �. As shown in Figure 1(a), we can define now �'#1,$ and �*++,$ as the boundaries of the 10% − 90% transition as �(�'#1,$) = 0.1 � and �(�*++,$) = 0.9 �, respectively. This provides an objective definition of the critical temperature �; ≡ 1/2(�*++,$ + �'#1,$) and the transition width ��; ≡ �*++,$ − �'#1,$, giving rise to �; = 12.1 ± 0.2 � and ��; = 0.3 ± 0.05� for the �CDC = 26 ± 0.5�� film presented in Figure 1(a). In a detailed study [21] we investigated NbN films with thicknesses �CDC = 4 − 20 �� as a function growth temperature �I$ and nitrogen partial pressure �CK. On such optimized films we established SSPDs employing a combination of electron beam lithography with a negative tone resist and reactive ion etching using a SF6/C4F8 plasma [22] [24] . Nanowire SSPDs were arranged in a meander-type fashion with typically 34× loops, nanowire widths 80 ± 10 ��, nanowire separation 170 ± 10 �� and a total length of 23 �� as shown in the scanning electron microscope images in the inset of Figure 1(b). In order to determine the the performance metrics of our SSPDs, we performed opto-electrical characterization measurements in a low-temperature (� = 4.3 ± 0.1 �) microwave probe station where individual devices can be contacted using mechanically adjustable microwave probes. A schematic circuit diagram of the setup used for probing the optical response of the detectors is shown in the inset of Figure 1(b). Hereby, the SSPD is operated just below the critical current �; using a bias-tee (1 �� inductance and 0.22�� capacitance) connected to a 100 �� resistor and a voltage source �D. Here, we assume
Details
-
File Typepdf
-
Upload Time-
-
Content LanguagesEnglish
-
Upload UserAnonymous/Not logged-in
-
File Pages14 Page
-
File Size-