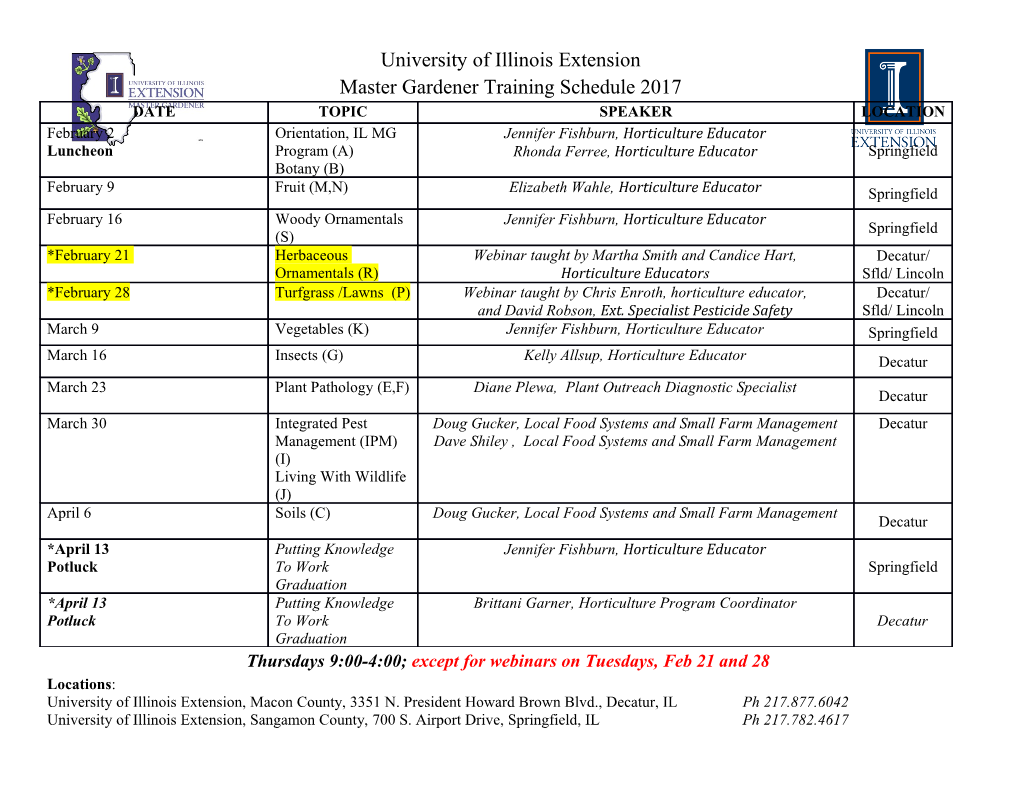
Ast 777: Star and Planet Formation Jonathan Williams, University of Hawaii Accretion disks https://www.universetoday.com/135153/first-detailed-image-accretion-disk-around-young-star/ The main phases of star formation https://www.americanscientist.org/sites/americanscientist.org/files/2005223144527_306.pdf Star grows by accretion through a disk https://www.americanscientist.org/sites/americanscientist.org/files/2005223144527_306.pdf Accretion continues (at a low rate) through the CTTS phase https://www.americanscientist.org/sites/americanscientist.org/files/2005223144527_306.pdf The mass of a star is, by far, the dominant parameter that determines its fate. The primary question for star formation is how stars reach their ultimate mass. Mass infall rate for a BE core that collapses A strikingly simple equation! in a free-fall time isothermal sound speed calculated for T=10K This is very high — if sustained, a solar mass star would form in 0.25 Myr… …but it can’t all fall into the center Conservation of angular momentum Bonnor-Ebert sphere, R ~ 0.2pc ~ 6x1017cm 11 Protostar, R ~ 3R⊙ ~ 2x10 cm => gravitational collapse results in a compression of spatial scales > 6 orders of magnitude! => spin up! https://figureskatingmargotzenaadeline.weebly.com/angular-momentum.html Conservation of angular momentum Angular momentum Moment of inertia J is conserved Galactic rotation provides a lower limit to core rotation (R⊙=8.2kpc, V⊙=220 km/s) Upper limit to rotation period of the star So how do stars grow? • Binaries (~50% of field stars are in multiples) • Lose the angular momentum through outflows • Collapse through a disk, not directly onto star https://www.eso.org/public/news/eso1916/ Disk size Can estimate size by balancing gravity vs centrifugal force Cons ang mtm ~ 100au This is sometimes referred to as the “centrifugal radius” Disk size Can estimate size by balancing gravity vs centrifugal force Cons ang mtm ~ 100au very sensitive to initial core size and angular speed (also ignores magnetic fields which add additional parameters that are not well measured) Numerical simulations on core-disk scales https://www.kuffmeier.com/movies Disk evolution over 1Myr https://www.kuffmeier.com/movies Accretion disk physics vKep Fg => radial shear, Ω ~ r-3/2 => any viscosity will lead to friction, heating, and radiation Accretion disk physics r1 r2 For each particle, the angular momentum and energy are Accretion disk physics The sum for both particles is If the particles move, J must remain the same (if no external torque acts on the system), but friction in the shearing disk means that E will decrease Accretion disk physics Viscous disks naturally spread out Pioneering paper: Lynden-Bell & Pringle 1974 Surface density evolution Lynden-Bell & Pringle 1974 Bath & Pringle 1981 Energy dissipation means the inner particle moves inwards; it loses angular momentum and the outer particle gains (J ~ r1/2) Ultimately, the inner particles fall, or accrete, onto the star. (the last step is along magnetic field lines) Accretion luminosity —> a (solar mass) protostar is bright, and readily detectable well before it begins nuclear fusion Accretion observations Class 0: fully embedded, can only use luminosity and kinematic measures of central mass Class I: infrared lines Class II: access to a variety of UVOIR diagnostics Recommended reading —> 2016, Annual Reviews Accretion observations Focus on Class II for now, as it is observationally accessible, though the star has reached ~99% of its final mass “Balmer jump” Balmer series —> relates to #H atoms and assuming free-fall onto stellar surface gives mass accretion rate Gas from the disk funnels along magnetic field lines, falling onto the surface in localized regions at ~ 300 km/s free-fall speed X-rays absorbed, re-radiated to produce UV-optical —> shocks —> X-rays continuum excess (—> “veiling”) and emission lines Hartmann et al. 2016 Accretion variability (a defining characteristic of T Tauri stars) Hartmann et al. 2016 Mass accretion rates vs stellar mass Determined from the excess continuum and/or emission lines (with considerable scatter) Hartmann et al. 2016 -8 Typical values ~ 10 M⊙/yr => M⊙/(dM/dt) ~ 100 Myr! => accretion rate must be ~102 x greater in the embedded (Class 0/I) phase But accreted mass over the ~2 Myr lifetime of Class II YSOs is 0.01M⊙ = 10 MJupiter => Class II disks have enough mass (and time) to form planetary systems! Mass accretion rates vs time (with considerable scatter) Hartmann et al. 2016 Unanswered questions - What sets the mass accretion rate? - Why does it vary as the square of the stellar mass? - Why does it decline approximately inversely with time? Inherently hard because of variability and much of the accretion luminosity comes out in the UV A short commercial break… ~1000 orbits (DDT) to take UV spectra of young stars + 255 hours VLT Large program + several other ancillary programs + Maunakea? Possibilities for student involvement (but needs funding…) Accretion rates of Class I protostars Expected to be higher than in Class II, because they have short lifetimes (~0.5 Myr) and the star needs to gain most of its mass before the envelope is lost. But hard to measure equivalent widths due to veiling. The warm envelope/disk adds a continuum to the stellar spectrum which decreases the line-to-continuum ratio and/or simply fills in absorption lines. Fλ Fλ Wλ Wλ λ λ => need high s/n, high spectral resolution near-infrared observations Accretion rates of Class I protostars standard stars Class I YSOs Template matching to get SpT => Teff HR diagram to get R★ Can also use NIR emission lines such as Brγ to estimate mass accretion rates Doppmann et al. 2005 Accretion rates of Class I protostars template + accretion observed accretion continuum template But we don’t generally find substantially higher accretion rates… Herczeg & Hillenbrand 2014 BLT diagram Huge scatter in Lbol, and tendency for slightly higher Lbol at lower Tbol (Class 0/I), but its not as strong an effect (~102) as simple core collapse theory predicts Dunham et al. 2014 The protostellar luminosity problem - first identified by Kenyon et al. 1990 - proposed that stars gain their mass in short bursts of high accretion and that we generally see YSOs in their quiescent state - alternative suggestion is that most of the low luminosity YSOs are actually proto-brown dwarfs (Offner & McKee 2011) - (This would imply that the protostellar mass function differ from the final mass distribution [stellar IMF] and requires the formation timescale to depend on mass. However, the observational situation is unclear - an interesting project would be to measure the Class II stellar mass distribution through a complete ALMA survey of a young star-forming region…) The protostellar luminosity problem Hartmann et al. 2016 Episodic accretion https://sites.lsa.umich.edu/lhartm/wp-content/uploads/sites/496/2017/05/mdotseq.jpg Episodic accretion - due to MRI / GI? Core collapse dumps material onto a disk at the centrifugal radius, but the disk cannot transfer material onto the star as fast as it acquires it - so it grows in mass until it becomes magnetically and/or gravitationally unstable Vorobyov et al. 2013 Are disks gravitationally unstable? Not apparent in the dust, but possibly in the gas? https://public.nrao.edu/news/alma-captures-stirred-up-planet-factory/ Huang et al. 2020 FUor outbursts Rarely seen, 8 since 1936 (=> greater than local SFR => stars must do this more than once) But observations are biased toward optical => late stages Hartmann et al. 2016 Infrared outbursts Notes: - this is not as luminous as a canonical FUor burst - 2MASS+UKIDSS comparison show outbursts are rare, once per ~104 years - ongoing JCMT monitoring project of Class 0/I sources is finding some, https://www.jpl.nasa.gov/news/news.php?feature=4518 small variability Safron et al. 2015 Further reading / viewing Hartmann, Herczeg, & Calvet 2016, Annual Reviews https://ui.adsabs.harvard.edu/abs/2016ARA%26A..54..135H/abstract.
Details
-
File Typepdf
-
Upload Time-
-
Content LanguagesEnglish
-
Upload UserAnonymous/Not logged-in
-
File Pages42 Page
-
File Size-