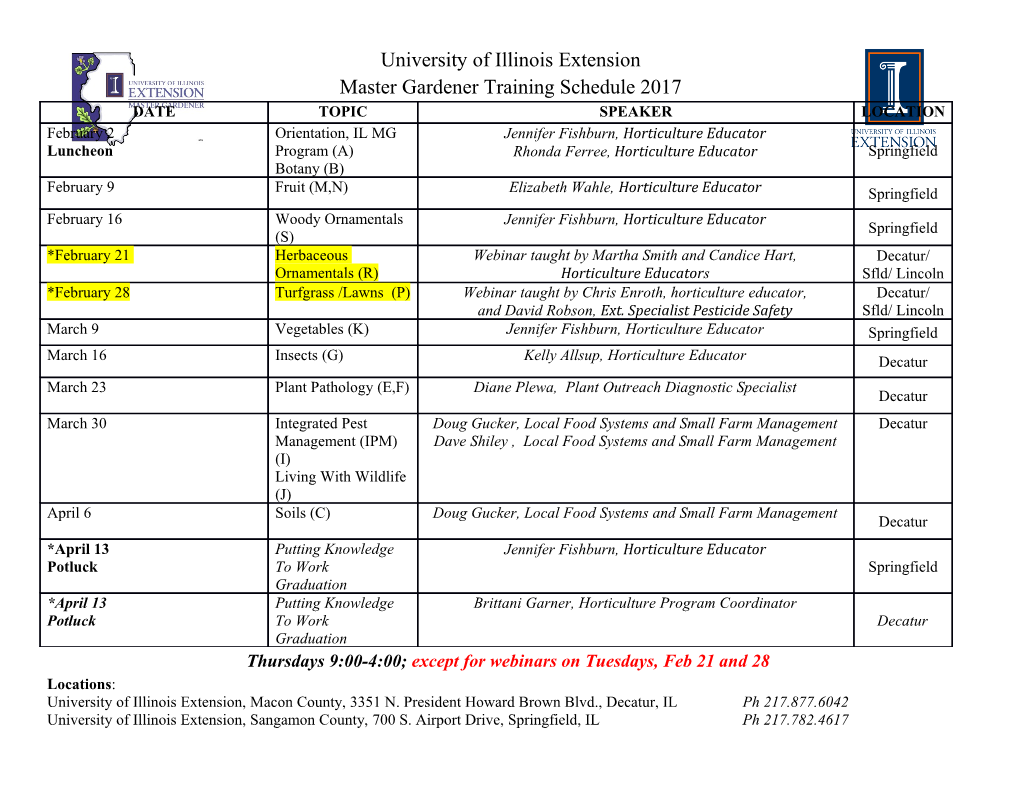
The Sun and its Restless Magnetic Field Manfred Sch¨ussler Max-Planck-Institut f¨urAeronomie Max-Planck-Str. 2, 37191 Katlenburg-Lindau, Germany [email protected] 1 Introduction The permanently changing magnetic field of the Sun is the ultimate driver of the various effects summarized under the labels solar-terrestrial relations and space weather. The aim of this paper is to give a brief overview of the physics of the solar magnetic field and its variability. This area of research is vast, so it would be pointless to aim at a comprehensive and detailed account in a paper of this format. So I restrict myself to a summary of the basic observational results and the currently prevailing theoretical models for the various processes. Reference is made to more detailed accounts whenever possible. More comprehensive representations of relevant topics can be found, for instance, in the books of Stix [1], Schrijver & Zwaan [2], Priest [3], Cox et al. [4], and Schmelz & Brown [5]. This contribution is organized as follows. After briefly presenting the gen- eral structure of the Sun in Sec. 2, various aspects of the solar magnetic vari- ability will be discussed in Sec. 3: magneto-convection (3.1), active regions (3.2), the global transport of magnetic flux on the solar surface (3.3), the 11/22 year cycle and its long-term modulation (3.4). Sec. 4 is devoted to the origin of the magnetic field and the activity cycle. We conclude with a brief outlook in Sec. 5. 2 The Structure of the Sun Schematically, the Sun and its extended atmosphere can be divided in a num- ber of layers according to the physical processes dominating the respective regions. Theoretical models of the solar interior, its pressure and temperature stratification as a result of hydrostatic equilibrium and the processes for the generation and transport of energy have reached a large degree of sophisti- cation. The validity of these models can be empirically tested through he- lioseismology, the analysis of the acoustic eigenmodes of the solar body [6]. Similar to the information about the Earth’s interior gained through the de- tection of seismic waves from earthquakes, the internal structure of the Sun can be reconstructed fairly precisely on the basis of the measured frequencies 2 Manfred Sch¨ussler of the acoustic eigenmodes excited by solar convection. The solar structure as derived through helioseiosmology is in very good agreement (at a level of 0.1%) with the theoretical models (see Fig. 1, left panel). Locally confined larger deviations, for instance directly below the convection zone, indicate that more subtle additional processes like gravitational Helium settling have to be taken into account. ∆ c2/ c2 Fig. 1. Left: Relative difference between the (squared) sound speed profile in the solar interior as inferred from helioseismology and a theoretical standard solar model [7]. Right: Solar rotation rate inferred from helioseismology as a function of radius at three solar latitudes. The formal errors are indicated by the shaded regions (both figures from from [8]). The core of the Sun (out to about 15% of the solar radius, R¯) is the do- main where thermo-nuclear reactions operating at temperatures above 107 K generate the solar energy flux. Most important is the proton-proton (pp) cycle, which (stepwise) generates 4He from hydrogen nuclei (protons). The neutrinos produced in the course of the nuclear reactions leave the core almost uninhibited and can be detected on Earth as direct witnesses of the energy- generating processes. The long-standing ‘solar neutrino problem’, resulting from the detection of much less (electron) neutrinos than expected from the nuclear reactions, found its resolution in the existence of neutrino oscillations which periodically change the ‘flavor’ of the neutrinos during their flight to Earth, so that only part of them are registered in the detctors [9]. This im- plies also a finite (albeit small) rest mass of the neutrinos and thus entails modifications of the standard model of elementary particles. The good agree- ment between helioseismic results and theoretical models of the solar interior indicated early on that the solar neutrino problem most probably would find its resolution from the side of particle physics, not solar physics. The energy generated in the core is transported outward by photons in the radiation zone of the Sun (often also called radiative interior), which covers the region 0.15–0:7 R¯. The photons are so often absorbed and re-emitted in The Sun and its Restless Magnetic Field 3 the dense solar matter that the transfer of energy through the radiation zone with its outward decreasing temperature can be very well be approximated by a diffusion process with a characteristic timescale of about 107 years. As the temperature decreases outward more and more, electrons start to recombine with the various atomic nuclei. The resulting possibilities for electronic transitions increase the opacity of the matter and thus decrease the diffusion coefficient for radiation, so that the temperature gradient has to grow steeper in order to keep the energy flux constant in a steady state. 6 Around 0:7 R¯ and at T ' 2¢10 K, the required temperature gradient for the radiative transport of the energy becomes steeper than the adiabatic temper- ature gradient. As a result, the stratification becomes unstable with respect to convective motions, which rapidly take over as the dominant mechanism of energy transport. The very good mixing properties of the convective motions lead to an almost adiabatic (isentropic) stratification of the convection zone, which spans the radius interval 0.7–1:0 R¯ [10]. Since convective motions can penetrate a certain depth into the stable radiation zone, a convective overshoot layer forms. The slightly subadiabatic (stable) stratification of the overshoot layer favors the storage of magnetic flux [11]. As we shall see in more detail Sec. 4.1, this is one of the reasons why the interface between the convection zone and the radiative core at about 0:7 R¯ is of particular interest for the generation of magnetic flux by the solar dynamo. A second indication comes from helioseismology, which can be used to infer the rotation rate in the solar interior as a function of depth and latitude. As shown on the right panel of Fig. 1, there is a layer of strong radial rotational shear (differential rotation) straddling the transition between the radiation zone and the convection zone [12]. Since differential rotation is one of the basic ingredients of virtually all models for the solar dynamo mechanism (which maintains the solar magnetic field in the first place), the discovery of this shear layer (the so-called solar tachocline) has important consequences for our understanding of the solar dynamo. The other ingredient for the solar dynamo are the convective motions of the electrically conducting solar plasma (see Sec. 4). The mass motions of solar convection can directly be observed in the form of granulation on the visible solar surface, which is the layer where the solar plasma becomes transparent for visible light. Fig. 2 shows the gran- ulation pattern between sunspots and pores, which are cooler and darker than their surroundings because their strong magnetic field suppresses the energy-carrying convective motions. The bright granules correspond to ris- ing hot gas while the network of darker intergranular lanes represents the downflow regions where the matter descends again into the convection zone after it has lost a substantial part of its internal energy through radiation into space. Apart from the dark sunspots and the granulation pattern, Fig. 2 also shows conspicuous bright points in the intergranular lanes: these rep- resent magnetic flux concentrations with field strengths similar to those in 4 Manfred Sch¨ussler sunspots (' 1500 Gauss = 0:15 Tesla, see [13]). Such magnetic elements owe their existence to the interplay between convection, radiation, and magnetic field commonly referred to as solar magneto-convection (cf. Sec. 3.1). Fig. 2. Granulation, sunspots, pores, and magnetic elements in the solar pho- tosphere as visible in the Fraunhofer G band at 430.4 nm, a wavelength range dominated by lines formed by the CH molecule. The magnetic flux outside the dark pores resides in tiny field elements located in the intergranular downflow re- gions. While larger magnetic structures are darker than the average photosphere, small-scale magnetic concentrations appear bright owing to the reduced abundance of CH molecules (and thus less absorption in the molecular lines) in their hot and tenuous atmospheres (image taken with the Dutch Open Telescope at the Roque de los Muchachos Observatory on La Palma, Spain; courtesy P. S¨utterlin). The kinetic energy density of convection, the thermal and the magnetic energy density are all of the same order of magnitude in the solar photosphere comprising the visible surface and the first few hundred km of the overlying solar atmosphere. Above the photosphere, the exponential decrease of gas density and pressure rapidly leads to a situation in which the magnetic field completely dominates the structure and dynamics. In the chromosphere and in the corona, the energy built up in the magnetic field by the interaction with convective motions in the lower atmosphere is released by various (not very well understood) processes like, for instance, shock dissipation, current sheet dissipation, and reconnection of magnetic field lines [14, 15]. As a conse- quence, the gas is heated and the temperature increases outward until coronal The Sun and its Restless Magnetic Field 5 temperatures of a few million K are reached. At the same time, magnetic en- ergy is also released in various kinds of large impulsive phenomena like flares, surges, or coronal mass ejections. Such events are the cause of the multitude of near-Earth phenomena and effects that travel under the signature ‘space weather’.
Details
-
File Typepdf
-
Upload Time-
-
Content LanguagesEnglish
-
Upload UserAnonymous/Not logged-in
-
File Pages26 Page
-
File Size-