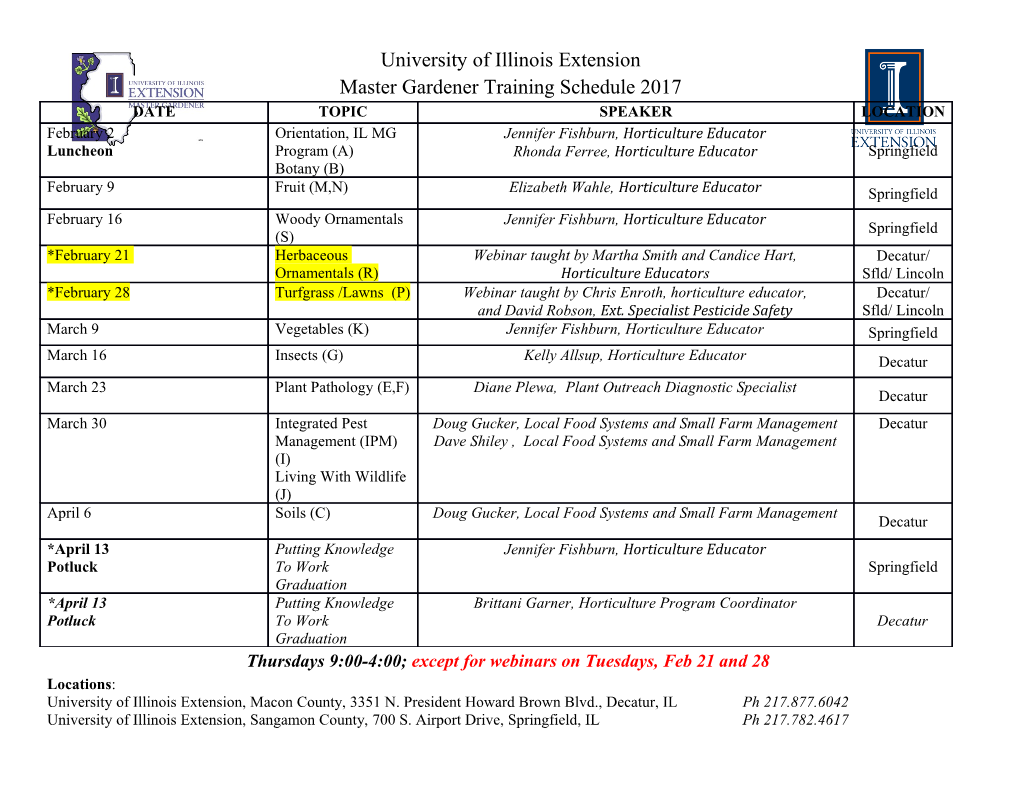
Article Multichannel Electrical Impedance Spectroscopy Analyzer with Microfluidic Sensors † Jaan Ojarand * Mart Min and Ants Koel Thomas Johann Seebeck Department of Electronics, Tallinn University of Technology, 19086 Tallinn, Estonia; [email protected] (M.M.); [email protected] (A.K.) * Correspondence: [email protected]; Tel.: +372-502-4723 † This manuscript is extension version of the conference paper: Compact Multichannel Device for Differential Impedance Spectroscopy of Microfluidic Sensors. In Proceedings of the 16th Biennial Baltic Electronics Conference (BEC), Tallinn, Estonia, 8–10 October 2018. Received: 1 March 2019; Accepted: 17 April 2019; Published: 20 April 2019 Abstract: Impedance spectroscopy is a common approach in assessing passive electrical properties of biological matter. However, several problems appear in microfluidic devices in connection with the requirement for high sensitivity of signal acquisition from small volume sensors. The developed compact and inexpensive analyzer provides impedance spectroscopy measurement from three sensors, both connected in direct and differential modes. Measurement deficiencies are reduced with a novel design of sensors, measurement method, optimized electronics, signal processing, and mechanical design of the analyzer. Proposed solutions are targeted to the creation of reliable point-of-care (POC) diagnostic and monitoring appliances, including lab-on-a-chip type devices in the next steps of development. The test results show the good working ability of the developed analyzer; however, also limitations and problems that require attention and further improvement are appointed. Keywords: impedance spectroscopy; microfluidic sensor; non-faradaic; front-end electronics; differential measurement; calibration; label-free detection; lab-on-a-chip 1. Introduction The current article is an extended revision of the recent conference paper [1], in which impedimetric microfluidic devices target facilitation of dielectric and conductivity measurements of small fluidic volumes. The measuring fluid may be an electrolyte cell suspension or microparticles, a biochemical solution of metabolites, proteins, and nucleic acids [2,3]. Most biosensors require a labeling substance attached to the target; during readout, the amount of labeling substance is detected, and it is assumed to correspond to the number of bound targets [4]. Labels can be, for example, fluorophores detectable with optical methods. Label-based methods are sensitive and widely used, but label-free sensors have several advantages, including simplicity and speed of the measurement procedure that enables real-time monitoring of the binding reaction, thus giving access to the kinetic and thermodynamic parameters [5]. Although optical label-free detection is introduced [5], electrical label-free biosensors are promising for point-of-care (POC) and home-diagnostics applications due to low cost and ease of miniaturization [6]. Most of the currently developed electrical biosensors use the faradaic process, where the charge is transferred across an interface and redox species are alternately oxidized and reduced by the transfer of an electron to and from the metal electrode (a redox process) [4,7,8]. Alternatively, a non-faradaic method has several advantages for POC applications [7]. The addition of redox species is not required in this case, and two electrodes are used instead of three. The need to add redox species makes the experiment more complicated because their properties and dosing accuracy also Sensors 2019, 19, 1891; doi:10.3390/s19081891 www.mdpi.com/journal/sensors Sensors 2019, 19, 1891 2 of 28 affect the measurement results. The use of the third (reference) electrode requires extra space in the sensor, and its properties (e.g., the stability) may reduce the accuracy of measurements significantly [9]. Moreover, the use of non-faradaic method allows shorter measurement time that provides better agreement with time invariance criterion of the system when monitoring time-dependent properties of the sample under test (SUT). The SUT is typically a small particle (e.g., cells, bacteria) in biocompatible solutions, e.g., in phosphate buffer solution (PBS). The presented analyzer and sensor uses the non-faradaic method that allows a simpler design of small volume measurement cells with two electrodes, overwhelming the drawbacks of the faradaic method with the three electrode sensors discussed above. The novelty of the proposed designs is related to the higher sensitivity of differential measurements with a capability to preserve actual impedance values, along with the design of the sensor with the 4-nanoliter volume of the measurement cell. The use of three direct and two differential measurement channels allows for sensitive enough comparison of properties of two SUT, with the third sample (the reference, which is typically clean PBS solution) used as a carrying medium for the first two channels. The description of the impedance analyzer introduces the structure of measurement circuitry, focusing mainly on its analog front-end part. The used analog-to-digital (AD) and digital-to-analog (DA) converters, digital input-output (IO), and communication parts, are all described only briefly since a commercial digital oscilloscope module was in use for that purpose. Several important topics related to the detection and measurement of small changes of electrical impedance spectra (EIS) in a fluidic environment are discussed as follows: • Main measurement methods and typical shape of spectra • Selection of excitation signal parameters (source type, frequency range, waveform, number of frequencies) • Calibration procedure and related issues • Short description of the software and user selectable parameters In the discussion section, the results of the EIS measurements of the test set-up are analyzed. The introduced test analyzer is not the final solution for POC applications. The final versions will be certainly simpler, smaller, and cheaper. However, despite the moderate cost and dimensions, it provides flexibility and sensitivity, suiting experiments in the process of designing dedicated devices. 1.1. Impedance Measurement Methods and Excitation Signal Sources To measure unknown impedance, we can apply a known voltage excitation across the object and measure the current flowing through it, or we inject a known current into that object and measure the voltage across. We can also measure both current and voltage simultaneously and calculate the impedance as their ratio. The last method is preferable if the excitation source does not generate a steady signal. This situation is typical for current sources at frequencies above 1 MHz, since their output impedance decreases because of stray capacitances and degradation of the performance of electronic components [3]. An example with a single cell in saline solution (Figure 1a) illustrates the formation of the impedance magnitude spectrum in the microfluidic sensor. The shape of the magnitude spectrum, measured by the aid of electrical impedance spectroscopy (EIS), typically has two decaying parts (Figure 1c). The first one is caused by so-called double layer capacitance that is formed near the surface of the electrode made of noble metals (e.g., gold or platinum). The second decaying part is introduced by the capacitance Cs of the liquid solution between the electrodes and by stray capacitances Cst formed from the input capacitance of both measurement electronics and the capacitance of connection leads and connectors. In the simplified electrical model presented in Figure 1b, the membrane resistance Rm is omitted, since its value is very high in comparison with solution resistance Rs and cytoplasm Rcy. The capacitance Cdl is introduced in the simplified model (Figure 1b) instead of a constant phase element (CPE) used in more accurate electrical models Sensors 2019, 19, 1891 3 of 28 discussed below. Note also that the detection of changes of R cy and Cm would require high resolution since they are masked by significantly lower impedances of Cs and R s connected in parallel. Typically, such design of sensors suits only for the characterization of mean values of a larger amount of cells. To address these issues, the detection area of the sensor should be small [10]. The novel design of the sensor (Section 2.2) provides a solution for that task. (a) (b) (c) Figure 1. A magnitude spectrum (c) of the impedance of a single cell in saline solution (a) is described by a simplified electrical impedance model (b). The values of components are: Cdl = 4 nF, Cm = 1 pF, Cs = 2 pF, Cst = 3 pF, Rs = 60 kΩ, and Rcy = 100 kΩ. Magnitude spectrum (c) of the cell impedance is measured, applying an excitation voltage Ve and measuring the response current Ir through it. This is converted into a voltage Vr using a transimpedance amplifier (TIA). In the case of using current excitation, the amplitude of the current must not produce a voltage drop above 50 mV on the double-layer impedance of electrodes to avoid significant nonlinearity of current-to-voltage relationship [3,4,11]. At higher frequencies, the use of a fixed (constant) current produces a low and decaying amplitude of the response voltage that decreases the signal to noise (SNR) of measurements. Note that in a higher frequency area (above 10 kHz in the current case) the voltage drop at the sensor is mostly determined by the resistance of the solution Rs and reactance of capacitances Cs and Cst. A constant voltage excitation Ve (see Figure
Details
-
File Typepdf
-
Upload Time-
-
Content LanguagesEnglish
-
Upload UserAnonymous/Not logged-in
-
File Pages28 Page
-
File Size-