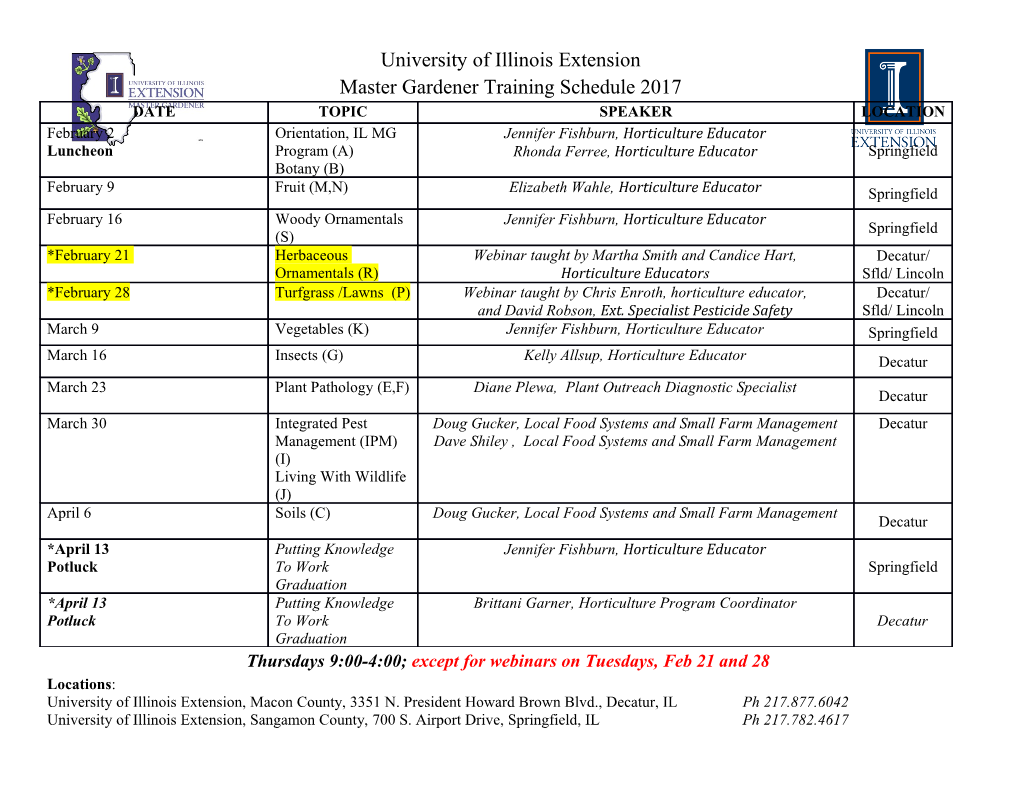
Microarray study of temperature dependent sensitivity and selectivity of metal/oxide sensing interfaces J. Tiffany, R. E. Cavicchi, S. Semancik National Institute of Standards and Technology Gaithersburg, MD 20899 ABSTRACT Conductometric gas microsensors offer the benefits of ppm-level sensitivity, real-time data, simple interfacing to electronics hardware, and low power consumption. The type of device we have been exploring consists of a sensor film deposited on a "microhotplate"- a 100 micron platform with built-in heating (to activate reactions on the sensing surface) and thermometry. We have been using combinatorial studies of 36-element arrays to characterize the relationship between sensor film composition, operating temperature, and response, as measured by the device's sensitivity and selectivity. Gases that have been tested on these arrays include methanol, ethanol, dichloromethane, propane, methane, acetone, benzene, hydrogen, and carbon monoxide, and are of interest in the management of environmental waste sites. These experiments compare tin oxide films modified by catalyst overlayers, and ultrathin metal seed layers. The seed layers are used as part of a chemical vapor deposition process that uses each array element's microheater to activate the deposition of SnO2, and control its microstructure. Low coverage (2 nm) catalytic metals (Pd, Cu, Cr, In, Au) are deposited on the oxides by masked evaporation or sputtering. This presentation demonstrates the value of an array-based approach for developing film processing methods, measuring performance characteristics, and establishing reproducibility. It also illustrates how temperature-dependent response data for varied metal/oxide compositions can be used to tailor a microsensor array for a given application. 1. INTRODUCTION As the power of computing microchips continues to improve, increasingly sophisticated, inexpensive data analysis tools are becoming available for chemical sensing technologies that gather complex data from the environment. Examples include chemometric and neural-network based pattern recognition algorithms, which enable the response from an array of non- chemically-specific sensors to be converted to useful information such as the concentration and composition of the components of a gas mixture1-5, or the match to an identified odor in an "electronic nose" application6-8. The demand for more detailed data in fields as diverse as process sensing, sensing for transportation and building air quality, etc., coupled with this computing capability, now places even more pressure on sensor developers to produce a greater variety of sensor types and materials to produce the required data. Similar to the challenges of the development of new catalysts and new pharmaceuticals, this situation is amenable to the use of combinatorial synthesis methods to efficiently search a multidimensional phase space for useful sensing materials. Of the many approaches available to develop a gas sensing technology, conductometric sensors offer the advantages of being a simple measurement, using robust materials, and providing good sensitivity, and fast response times. For example, a nanoparticle tin oxide film was shown to have sub nanogram/gram (ppB) sensitivity to methanol in air and produced a stable response to over 100 hours of gas-on / gas-off cycling9. Conductometric sensors may be fabricated from a variety of materials combinations, including conducting polymers such as polypyrole10 or polythiophene11 or carbon-loaded polymers12, and metal oxides with or without catalysts13-18. The sensing materials often exhibit strong temperature-dependent properties, which can enhance the sensitivity to a particular gas, and change the relative sensitivities to a set of different gases. Metal oxide sensing materials have been fabricated for decades as sintered pastes of ceramic material surrounding a platinum heater operated at temperatures in the range of 200 oC to 400 oC. New materials are developed by fabricating discrete devices, one by one, and evaluating their performance in a gas testing system. Miniaturized versions of the discrete conductometric sensors were developed by combining microelectromechanical systems (MEMS) and complementary metal oxide semiconductor (CMOS) technologies to produce a testing platform termed a "microhotplate19." These devices are fabricated in silicon, using the layer sequences available to CMOS technology, typically consisting of silicon oxide, polycrystalline silicon (polysilicon), and a metal layer (aluminum, tungsten) and then subjected to a surface-micromachining etch step, which produces a pit underneath suspended layers of these materials. A typical device size is 100 µm. The pit provides a high degree of thermal isolation, allowing the suspended portion of the devices to achieve the high temperatures (>500 oC) needed for controlling the sensor material microstructure as well as activating certain sensing reactions. In addition, the thermal isolation and miniaturization allow the devices to operate at these temperatures with relatively low power consumption (tens of milliwatts), making battery-operated devices possible. The small size also results in a rapid thermal time constant, so the heaters can be switched to different temperatures in a few milliseconds, which opens up new sensing modes based on dynamic temperature programming20-21. Most importantly, the batch fabrication used to produce the CMOS layers can be readily employed to fabricate arrays with hundreds of microhotplates. For sensors, the limit on array size is set by practical lead counts to get signals in and out. For example, a 48-element array can be produced with 98 leads: 48 heater drivers, 48 sensing highs and two commons. In the future, on-chip multiplexing will eliminate this practical restriction. Previously we have described the use of a 36-element array to investigate the effect of different seed layers on the microstructure of chemical vapor deposited tin oxide22. In the work described here, we use 4 and 36 element arrays to survey the effects of some different catalyst materials added to the top surface of tin oxide for a set of nine test gases. Although the larger arrays require more process steps to produce a variety of materials over the array, once fabricated, they allow simultaneous testing to be easily performed. When considering new test materials, we have found the four-element arrays are useful because of the reduced fabrication time, and reduced risk of a single process step spoiling the entire array. The trade-off here is the increase in effort and time required to test only four elements at a time, and the larger number of factors from fabrication and test conditions that can affect reproducibility. The goal of these experiments is to demonstrate a combinatorial approach for developing a database that will relate the effect of the catalyst additives to the gas sensitivity for different gases. Such a database might eventually be used as a lookup table in which a sensing need is identified, a promising materials combination is selected from the database, and additional experiments are performed to further customize the material, and its use (operating temperature or temperature program) for the application. 2. EXPERIMENT The basic microhotplate device configuration is illustrated schematically in Figure 1. The device consists of a polysilicon heater, a metal heat distribution plate, and metal contacts separated by layers of insulating SiO2. The devices were fabricated at Lincoln Laboratories at the Massachusetts Institute of Technology23 using mask designs configured at NIST. The metal layers for this process consisted of a sandwich of titanium/ aluminum/ titanium-tungsten. The fabrication process used chemical-mechanical polishing between layers, to produce a smooth deposition surface with a root mean square roughness of 1.1 nm as determined by atomic force microscopy. Figure 1. Schematic of layers within a microhotplate. Wafers received from the fab were diced into chips. Chips were subjected to an anisotropic silicon etch consisting of either ethylenediamene-pyrochatechol water or tetramethylammonium 100 µm 340 µm Figure 2. 4-element array of microhotplates. Figure 3. 48-element array of microhotplates. hydroxide. If formulated correctly, the latter etch is less aggressive on exposed metal layers. Examples of a four-element and a 48-element array are shown in Figures 2 and 3. The etched chips were packaged in 40-pin dual-in line packages (4-element array) and 84-pin packages (48-element array.) Only 36 of the 48 elements were used. Tin oxide was grown by chemical vapor deposition (CVD) onto a nickel-seeded surface. The nickel seed layer, 1.6 nm thick, speeds the nucleation process and produces a finer grain tin oxide film as compared to films grown on an unseeded hotplate. The seed layer was prepared by the vacuum evaporation of nickel onto the entire array. The CVD process was performed in a cold-wall system operating at about 500 Pa. The sources were produced by flowing argon through a tetramethyltin bubbler operating at temperature of -40 oC at a flow rate of 20 standard cubic centimeters per second (sccm), flowing oxygen at 300 sccm, and using a balance of argon flowing at 300 sccm. The CVD process uses the microheater of an array element to provide the thermal energy for decomposition of the precursor, and reaction with oxygen to grow tin oxide on that element24- 26. Each array element was monitored to record the electrical resistance of
Details
-
File Typepdf
-
Upload Time-
-
Content LanguagesEnglish
-
Upload UserAnonymous/Not logged-in
-
File Pages8 Page
-
File Size-