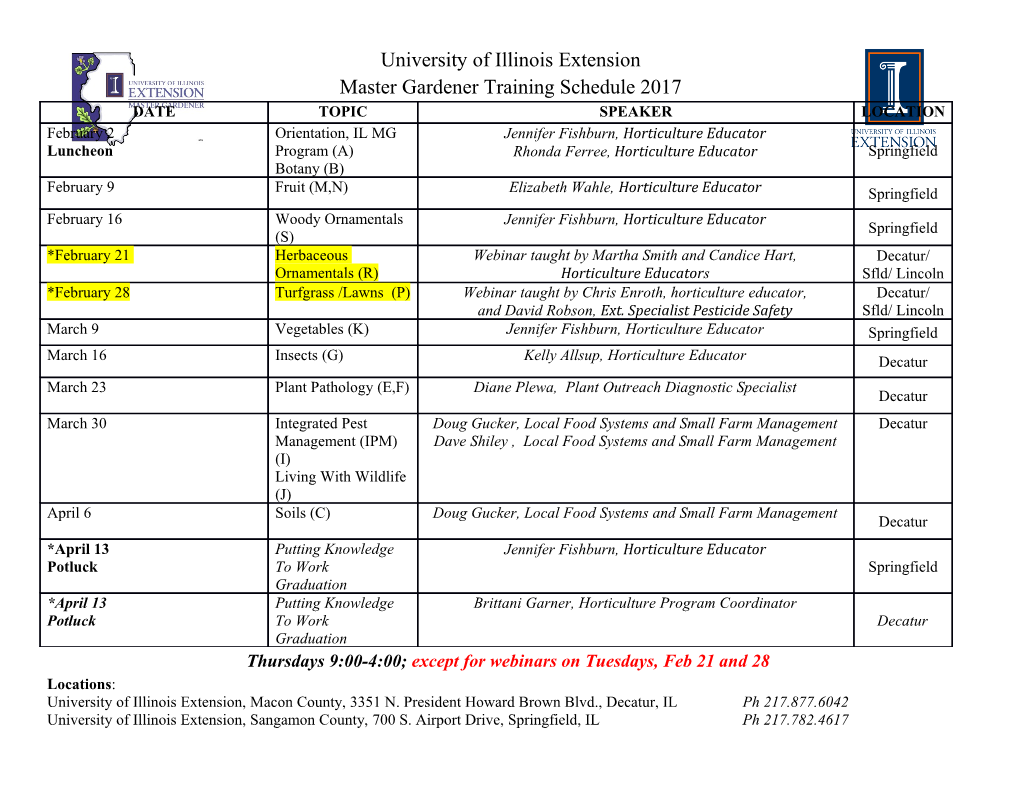
UvA-DARE (Digital Academic Repository) Isovaleric acidemia: an integrated approach toward predictive laboratory medicine Dercksen, M. Publication date 2014 Link to publication Citation for published version (APA): Dercksen, M. (2014). Isovaleric acidemia: an integrated approach toward predictive laboratory medicine. General rights It is not permitted to download or to forward/distribute the text or part of it without the consent of the author(s) and/or copyright holder(s), other than for strictly personal, individual use, unless the work is under an open content license (like Creative Commons). Disclaimer/Complaints regulations If you believe that digital publication of certain material infringes any of your rights or (privacy) interests, please let the Library know, stating your reasons. In case of a legitimate complaint, the Library will make the material inaccessible and/or remove it from the website. Please Ask the Library: https://uba.uva.nl/en/contact, or a letter to: Library of the University of Amsterdam, Secretariat, Singel 425, 1012 WP Amsterdam, The Netherlands. You will be contacted as soon as possible. UvA-DARE is a service provided by the library of the University of Amsterdam (https://dare.uva.nl) Download date:02 Oct 2021 Chapter 1 General introduction and outline of thesis 1 Chapter 1 1 Preface Organic acidemias are an important group of inborn errors of metabolism (IEMs), which mainly involve defects in the catabolism and related intermediary pathways of carbohydrates, amino acids and fatty acids. Some organic acidemias can be treated with a relatively high success rate, but this may depend on rapid diagnosis and early treatment interventions. Treatment is in general aimed to reduce metabolic decompensation as well as to prevent neurological damage and developmental delay. The phenotypical presentation of the organic acidemias is broad. Isovaleric acidemia (IVA), which is the result of the defective isovaleryl-CoA dehydrogenase (IVD) in the catabolism of leucine, a branched chain amino acid (BCAA), was one of the first organic acidemias to be described, almost 50 years ago by Tanaka et al. (1966). Subsequently, Budd et al. (1967) clarified the primary clinical manifestations of the disease. A large number of case studies have progressively emphasized the clinical heterogeneity of IVA (reviewed by Ensenauer et al., 2004; Vockley and Ensenauer, 2006). These reviews indicate that the original notion of a one genotype, one phenotype relationship is probably the exception rather than the rule, because the disorder is accompanied by neurological aberrations in varying degrees (Budd et al., 1967; Grünert et al., 2012). The clinical variability, even in patients with the same genetic background, as well as novel diagnostic biochemical tools and expanding treatment possibilities for isovaleric acidemia, served as main motivation for this study. A timely diagnosis of IVA, through newborn screening (NBS) programs around the world has improved the clinical outcome of most patients by the early implementation of treatment. The development of mass spectrometry for NBS has led to the identification of IEM patients with an unexpectedly wide clinical presentation (Ensenauer et al., 2004; Dionisi-Vici et al., 2006). Recent investigations of IVA patients in Korea, Taiwan and Thailand have further emphasized the diversity of genotype-phenotype relationships in different populations and even within families (Lee et al., 2007; Lin et al., 2007; Vatanavicharn et al., 2011). IVA is periodically diagnosed in the Caucasian South African population (although its prevalence is still unknown). The molecular and biochemical characteristics, however, have been poorly documented thus far. Consequently, the characterization of IVA in the South African population was one of the main aims of this study. Nutritional and pathological aspects were also subjects of investigation. A crucial additional factor in the conduct of the research reported in this thesis is the advent of metabolomics, which is a highly sensitive, data-driven technology responsible for novel multidisciplinary approaches (as will be described in section 6 of this chapter) that permit the interpretation of pathophysiological aspects of disease. The metabolomic approach has also irrefutably led to the first proof-of-concept studies in IEMs, namely 2 General introduction and outline of thesis defects in the metabolism of propionate (Wikoff et al., 2007) and respiratory chain disorders (Smuts et al., 2012). The study of the metabolome and its changes in response to different physiological and genetic processes already shows promise in the identification of the mechanism of disease and of underlying problems associated with such disorders, for example pathological effects and nutritional deficiencies. A metabolomic analysis may ultimately be able to determine the choice of therapy, recognize susceptible patients and predict possible toxic effects of treatment (McCabe, 2010; Mamas et al., 2011). An exciting further spin-off may be the follow-up investigations of the disease which will ultimately lead towards predictive laboratory medicine. The recognition of the unique potential of metabolomics in the investigation of IEMs therefore became one of the aims of this investigation, as will be elaborated on in this chapter. The following sections address topics that have a close bearing on the study reported in this thesis. The initial discussion will outline the metabolism of branched chain amino acids (BCAA) in general, and present an overview of IVA. 2 Metabolism of branched chain amino acids The branched chain amino acids (BCAA) leucine, isoleucine and valine, are essential biomolecules which play a vital role as building blocks in protein synthesis. These neutral aliphatic amino acids, containing methyl-branched side-chains are present in protein-containing food. BCAAs are metabolized predominantly in the skeletal muscle and the liver (>70%). Between 8 – 30% of the oxidation of BCAAs may also take place in kidney -, brain -, heart -, pancreatic -, intestinal - and adipose tissue (Suryawan et al., 1998). The degradation of these compounds results in the formation of important metabolites, which are vital in biochemical processes (e.g. in the biosynthesis of lipids), as well as in energy production (via the Krebs cycle and ketone body production). The degradation of the BCAAs starts with their initial transportation into the cell via a distinct L-amino acid-specific transporter, most likely Na+-independent (Shotwell and Oxender, 1983). The initial transamination step, identical for all three BCAAs, takes place in the cytosol via branched chain aminotransferase. Alternatively, BCAAs can also be transported into the mitochondria (with a neutral amino acid carrier protein) and converted into the corresponding branched chain keto acids (BCKAs) via mitochondrial branched chain aminotransferase. Both aminotransferases are pyridoxal phosphate- dependent and simultaneous amination of 2-ketoglutarate takes place to form glutamate. The aminotransferase step for leucine, isoleucine and valine is not individually regulated; however, the catabolism of each of the BCAAs is highly regulated by both allosteric and covalent mechanisms (recently reviewed by Chuang et al., 2012). 3 Chapter 1 The branched chain keto acids will have to cross the mitochondrial membrane through the action of a keto acid transporter. Once inside the mitochondria, all three branched chain keto acids are oxidatively decarboxylated via the branched chain keto acid dehydrogenase (BCKAD) complex to form branched chain acyl-CoAs. BCKAD is a multi-enzyme complex consisting of three subunits, namely: E1, a thiamine pyrophosphate-dependent decarboxylase; E2, a lipoamide acyltransferase; and E3, a FAD- and NAD-containing dihydrolipoyl dehydrogenase (Chuang et al., 2012). The sequential metabolic steps for each branched chain acyl-CoA differ and are illustrated in Fig. 1. In view of the focus in this thesis on isovaleryl-CoA dehydrogenase deficiency, this overview will deal mainly with the leucine degradation pathway (Fig. 1). Leucine is transaminated to 2-oxoisocaproic acid, which is subsequently converted to isovaleryl-CoA. Isovaleryl-CoA is resistant to β-oxidation, because of the methyl-group at the carbon-3 position and instead undergoes a carboxylation step catalyzed by biotin- dependent 3-methylcrotonyl-CoA carboxylase (3-MCC) to form 3-methylglutaconyl-CoA. The latter is hydrated by 3-methylglutaconyl-CoA hydratase to form 3-methyl-3- hydroxyglutaryl-CoA, which is followed by a lyase step [3-hydroxy-3-methylglutaryl-CoA lyase (HMG-CoA lyase)], resulting in the formation of acetyl-CoA and acetoacetate. The ketone body acetoacetate can readily be converted into the other ketone body D-3- hydroxybutyric acid. Both ketone bodies are produced in the liver and can be transported to peripheral tissues as an alternative energy source. The catabolism of leucine and several defects in this pathway have been reassessed recently by Vockley et al. (2012). Isovaleryl-CoA dehydrogenase deficiency will be discussed in detail in this chapter. Some aspects of the BCAA metabolism do not fall within the scope of these investigations, but are mentioned in relevant sections of this thesis. 4 General introduction and outline of thesis Fig 1: The catabolic pathways of BCAAs with special focus on the degradation of leucine (highlighted in blue) (Vockley et al., 2012). Numbers indicate the enzymatic steps within the catabolic pathways: 1) branched
Details
-
File Typepdf
-
Upload Time-
-
Content LanguagesEnglish
-
Upload UserAnonymous/Not logged-in
-
File Pages45 Page
-
File Size-