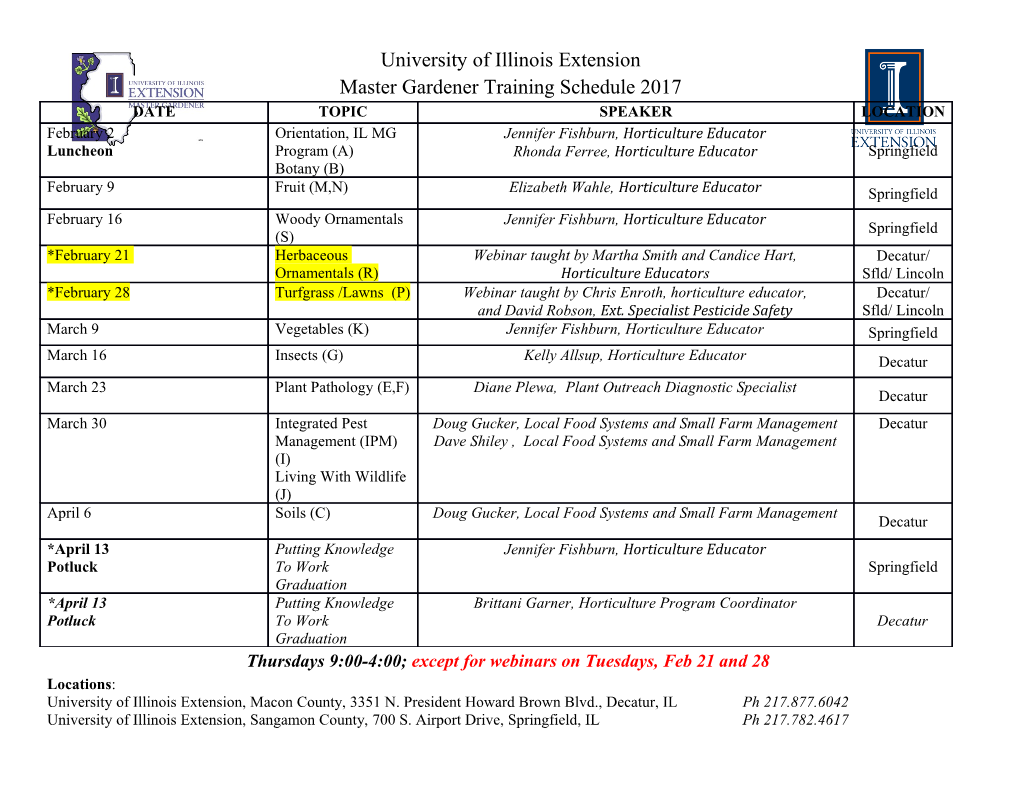
<p> General Genetics Biology 290 Chapter 12 Reading Guide</p><p>Overview: We skip ahead this week to follow-up on our thinking about RNA with an exploration of the myriad of ways that eukaryotes can regulate gene expression. This is a huge and fascinating topic! Remember that this is an essential challenge for multicellular life: How do you create all these different body parts from a single genome? In addition, we’re learning more and more about the critical role gene expression patterns play in human disease. It’s great stuff. Jump in!</p><p>An Overview of Transcriptional Gene Regulation: We’ll consider two broad categories of gene regulation in this chapter, transcriptional regulation and post-transcriptional regulation. Transcriptional regulation is well studied; it typically involves a molecular signal that causes regulatory proteins to bind to specific sites on DNA, controlling the rate of transcription of particular genes. </p><p>This is especially effective in eukaryotes because of the larger size and greater complexity found in our RNA Polymerase II. Our ‘default’ position, unlike the prokaryotes, is to have our genes turned off. Several key steps have to happen to properly initiate transcription. Typically, a series of more generalized transcription factors have to bind at nearby promoter-proximal elements. A second set of more specific transcription factors must also bind at distantly located sequences known as enhancers. In the image at right you can see how the DNA loops around, allowing the RNA polymerase to interact with the transcription factors binding to these enhancers. Note that both these binding sites are distinct from the promoter! (The promoter can be recognized in this image by the presence of the TATA box!)</p><p>Lessons From Yeast: We’ll spend some time exploring what’s been learned from the GAL system in yeast, the E. coli of the eukaryotic world. The GAL system is a set of carefully regulated genes that allow yeast to metabolize the sugar galactose. Several genes are required to produce these enzymes, and a second set are required for regulating the expression of the enzyme genes. Whew! Clearly the yeast needs to sense and respond to levels of galactose in its environment, producing these proteins when it’s present, and conserving energy when it’s not. </p><p>How can this possibly work? The secret is the Gal4 protein. Gal 4 binds at specific enhancer sites (also called upstream activation sequences), as shown at left. Once bound, a separate domain of the Gal4 protein, the activation domain, can bind either an inhibitory protein (Gal80) or, in the presence of galactose, help activate transcription of the GAL genes. There’s lots of great experimental evidence that supports this model. We’ll talk through more of it in class!</p><p>Despite their diminutive nature, yeast have alos been great models for examining cell-type regulation involving whole sets of genes. This works because yeast can exist in either or two haploid cell types (a and αlpha) or as a diploid form (a/alpha). This has huge implications in their lifecycle as an alpha cell will only mate with an a cell due to the production of a pheromone that triggers cell arrest. (The diploid cell type doesn’t mate.) How do these cells control the expression of different sets of genes in each of these three types?</p><p>Analysis of mutants has shown that the key lies in the MAT locus. Two alleles are found at this locus; one allele causes the expression of the structural genes of an a-type cell while the other triggers the expression of the alpha-type specific genes. There are, of course, a few other proteins involved. We’ll take a closer look at them in class. Chromatin! One of the big differences between prokaryotes and eukaryotes is the presence of chromatin. Remember that eukaryotes package their DNA coiled around clusters of eight histone proteins; this cluster is called a nucleosome. This packaging is critical because it means that most of your DNA is not accessible for transcription. The histones are in the way! Thus chromatin remodeling provides a powerful way to regulate gene expression. Even more fascinating, the structure of your chromatin, and thus your pattern of gene regulation, can be inherited. We call this type of inheritance epigenetic inheritance. If you haven’t studied epigenetics before, you’re in for quite a shock!</p><p>So how does this all work? There are several key mechanisms. This simplest is chromatin remodeling. In this process the histone octamer slides, exposing, or hiding, specific genes, as shown at right. Another key mechanism involves changes in the functional groups attached to the histone tails. One of the most common modifications is the attachment of an acetyl group to the tail. Acetylation relaxes the chromatin, potentially activating gene expression. Deacetylation removes the acetyl groups, causing the chromatin to condense, blocking transcription. The addition of acetyl groups is catalyzed by the enzyme histone acetyltransferase (HAT). You can probably guess what the histone deacetyltransferase (HDAC) does!</p><p>In addition to these heritable patterns of acetylation on your histones, your DNA can also be modified by the attachment of methyl groups, a process known as DNA methylation. (Patterns of DNA methylation are also heritable!) In mammals, the methyl group is typically added to the cytosine in a CG pair. Most of these sites are methylated with the exception of clusters of unmethylated CG pairs known as CpG islands. These CpG islands are clustered near promoters, suggesting that methylation typically silences genes.</p><p>Enhanceosomes: Welcome to the world of “omes”! The methods we’ve looked at so far typically result in genes being off or on. The reality, however, is that we really need finer control (a volume control, if you will). We can adjust the level, or volume, of transcription through the clustering of transcription factor binding sites in the enhancers. This allows several transcription factors to bind to adjacent sites, creating an enhanceosome. This produces a synergistic response, upregulating the level of transcription. As the enhancers that facilitate all this upregulation can be located thousands of bases away from the gene of interest, there is some risk that the wrong gene will be amplified. Research suggests that special proteins called enhancer blocking insulators have evolved to prevent this. </p><p>Long-Term Inactivation of Genes: We’re back to yeast to explore mechanisms through which organisms can more permanently silence genes. The key here is to remember the difference between the highly condensed regions of heterochromatin, and the less dense euchromatin. Remember that chromatin packing changes regularly throughout the cell cycle. In addition, some regions tend to stay condensed, especially those around the telomeres and centromeres. As you might imagine, most of our genes are concentrated in euchromatin.</p><p>This understanding of chromatin packaging led to a fascinating discovery in fruit flies. A geneticist observed some very unusual flies that had patches of both white and red colors within a single eye. A closer look showed that a chromosomal rearrangement had occurred. This rearrangement placed the white gene adjacent to a region of condensed heterochromatin near the centromere. In some cells, the heterochromatin had spread, silencing the white gene!! The existence of patches also demonstrated that cells produced mitotically from the cells whose heterochromatin had spread inherited the expanded heterochromatin! </p><p>As you might imagine, this crazy discovery created the perfect opportunity to look for mutations that either enhanced or suppressed this phenotype. Identifying the mechanism of these mutants provided some powerful insights on the process of chromatin condensation. It also lead to the discovery of barrier insulators; these molecules prevent the spread of heterochromatin, protecting regions containing important genes.</p><p>Imprinting: Genomic imprinting occurs when one copy of a particular gene is silenced. In maternal imprinting, the copy from mom is inactivated. In paternal imprinting, dad’s copy is silenced. This occurs without any changes to the DNA sequence! Thus two organisms can have dramatically different phenotypes even though they have identical genomes. This occurs, we think, because of specific, heritable patterns of DNA methylation. The presence of methyl groups on the DNA blocks the binding of transcription factors and other proteins from binding to the DNA and launching transcription.</p><p>X-Chromosome Inactivation: As you’re probably aware, mammalian females are in the awkward position of having two copies of every gene on the X chromosome. This creates a potential problem for gene dosage. Thankfully, one X chromosome is silenced, correcting for the “ double dose”. You can often see this condensed, silent X chromosome in the nucleus of cells, forming a structure called a Barr body (shown at the arrow in the DAPI stained nucleus at right). This inactivation occurs because of the activity of a non-protein coding RNA (ncRNA) called Xist. The mechanism is still unclear, but Xist seems to coat the chromosome that produces it, triggering condensation. miRNA: Last but not least, don’t overlook the role that miRNA can play in gene regulation. As we learned in chapter 8, these non-coding transcripts get cleaved into smaller, active miRNA that bind to RISC, inhibiting translation in a sequence-specific manner.</p>
Details
-
File Typepdf
-
Upload Time-
-
Content LanguagesEnglish
-
Upload UserAnonymous/Not logged-in
-
File Pages3 Page
-
File Size-