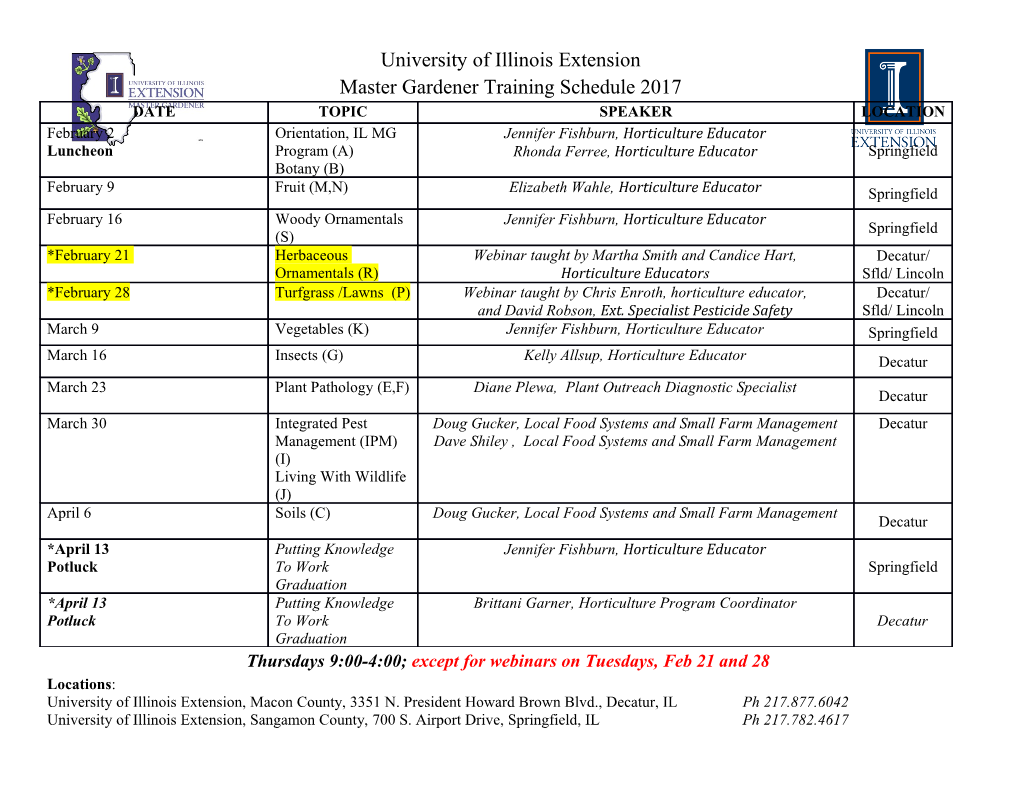
<p> 8.1 Launch, Outbound Secondary, and Return Power Patrick Kennedy</p><p>Nomenclature A = area, m CTV = crew transfer vehicle d = degradation factor dd = depth of discharge ERA = Earth return assembly ERV = Earth return vehicle Hab = habitation module M = mass, kg NTR = nuclear thermal rocket P = power, W RFC = regenerative fuel cell RTG = radioisotope thermoelectric generator t = time, s V = volume = efficiency = solar radiation flux, mW/cm2</p><p>8.1.1 Introduction Each phase of the PERForM mission from Earth launch until the landing on Mars requires power. In this chapter we discuss the power requirements during each phase and the power systems designed to meet those requirements.</p><p>8.1.2 Considerations For power generation, we consider solar arrays, radioisotope thermoelectric generators (RTG), regenerative fuel cells (RFC), and batteries, as well as the possibility of drawing power from the nuclear thermal rockets (NTR). Solar arrays are a proven technology and solar energy is available throughout the transit, but the required array is large. RTGs are another proven technology, however the quantity of plutonium fuel necessary to meet the required power levels poses significant availability concerns. Fuel cells are also proven in space flight, however the amount of fuel needed for primary fuel cells is prohibitive. This drives our consideration toward RFCs. They meet the power requirements,</p><p>8-1-1 but require another power system for recharging. Batteries also meet the power requirements, but they are very massive. Since the NTRs are already being taken along during transit, drawing power from them is favorable. However, this is a new technology without demonstrated reliability on spacecraft.</p><p>8.1.3 Hab Launch/ERA Mars Landing Power The power system for launch and landing, if different from the primary power source during transit, will serve as a backup during transit. Because of this, a 20 kW design is required. This provides ample power during the launch and landing stages of the mission as well as sufficient power to run emergency equipment. Solar arrays cannot be used during these stages of the mission. During launch, the NTRs are used for propulsion and are not available for power generation. Also, they are shed when the tether is released before landing on Mars. Since the Hab will temporarily be in orbit around Mars and this system needs to provide power during that orbit, batteries are exceedingly massive. RFCs provide the necessarily power at a lower mass than batteries. Since they are rechargeable, less fuel needs to be carried than if we reject the waste products. For these reasons, we choose regenerative fuel cells for the power source during the Hab launch and landing. We choose a fuel cell using hydrogen and oxygen as the fuels. First, it is a tested system. A similar system has operated on the space shuttle with a demonstrated 99% availablility.1 Also, the waste product is water. Water is easily electrolyzed back into the hydrogen and oxygen fuels. We size the fuel cells from a curve-fit of data for RFCs. 2 Figure 8.1.1 shows the mass of the RFCs as a function of days of continuous operation. Highlighted in the figure is our design for 20 kW at five days of continuous operation as well as a design for 30 kW. We design for five days to provide enough power for the Martian landing as well as ample time on the ground to connect to the nuclear reactor. Figure 8.1.2 shows the total volume of the RFC system as a function of days of continuous operation. These plots show that as the duration for which the RFCs are required to provide power increases, their</p><p>8-1-2 mass and system volumes increase dramatically. Thus, we want to keep the total days of continuous operation as small as possible. Reducing the power output also results in a reduction in both the mass and volume of the system. Additionally, reducing the power output of the RFCs reduces the power necessary to recharge them. This allows us to reduce the size of the power system that provides the power for regeneration. This system is discussed in the next section.</p><p>Fig. 8.1.1 Plots of RFC mass as a function of days of continuous operation. [P. Kennedy]</p><p>8-1-3 Fig. 8.1.2 Plots of total RFC system volume as a function of days of continuous operation. [P. Kennedy]</p><p>With this design, our RFCs have a total mass of 3300 kg and a total system volume of 6.6 m3. This includes 3 separate fuel cells for redundancy. Each one of these cells is capable of providing all of the necessary power by itself. This system requires 47.5 kW of power to recharge the fuel cells.</p><p>8.1.4 Hab Transit Power The power system for the Hab in transit must provide power for, among other things, communication, reaction control thrusters, onboard avionics and other electronics, and human factors such as life support. Power requirements we received from other groups are: human factors requires 9 kW of power, communication nominally requires 1 kW, avionics requires almost 4 kW, and the 20 RCS thrusters require 30 W each for a total of 0.6 kW. This gives a total power requirement of 14.6 kW. To account for other power requirements that</p><p>8-1-4 may not have been taken into consideration, the transit power system is designed for 30 kW. This system must also provide the power to recharge the RFCs. This is the largest power requirement and provides the maximum power output requirement for this system. As previously discussed, RFCs providing long term power are both large and massive. Batteries have the same problems. Solar arrays providing the needed power are large, but solar power is a proven design. Drawing power from the NTRs is an unproven technology, but they are already being carried in transit. For these reasons, we choose drawing power from the NTRs as the primary Hab transit power and solar arrays as the first backup. The system to obtain power from the NTRs is discussed in the next chapter. The equation for finding the necessary area of a solar array is given by d * P A (Eq. 8.1.1)3 For this analysis, it was assumed that 5% of the array is eroded over its life,3 so d=0.95. The efficiency is 24%,4 so =0.24, and =58 mW/cm2 at Mars. Figure 8.1.3 shows the solar array area as a function of power required. Marked on the plot is our design as well as a comparison to show what array size would be needed if the RFCs provided 30 kW of power.</p><p>8-1-5 6 0 0 3 0 k W D e s i g n O u r D e s i g n 5 0 0</p><p>4 0 0 ) 2 m (</p><p> a e</p><p> r 3 0 0 A</p><p> y a r r A 2 0 0</p><p>1 0 0</p><p>0 0 1 0 2 0 3 0 4 0 5 0 6 0 7 0 8 0 A r r a y P o w e r P r o d u c e d ( k W )</p><p>Fig. 8.1.3 Solar array area as a function of necessary power production. [P. Kennedy]</p><p>The density of our array blanket is 1.2275 kg/m2.4 The blanket usually is 45% of the mass of the array3, but since our system is under artificial gravity, we have assumed that the blanket is only 20% of the total system mass. Figure 8.1.4 shows the solar array mass as a function of power required. </p><p>8-1-6 3 5 0 0 3 0 k W D e s i g n O u r D e s i g n 3 0 0 0</p><p>2 5 0 0 ) g k (</p><p>2 0 0 0 s s a M</p><p> y 1 5 0 0 a r r A</p><p>1 0 0 0</p><p>5 0 0</p><p>0 0 1 0 2 0 3 0 4 0 5 0 6 0 7 0 8 0 A r r a y P o w e r P r o d u c e d ( k W )</p><p>Fig. 8.1.4 Solar array mass as a function of necessary power production. [P. Kennedy]</p><p>With this design, our solar arrays have a total mass of 2000 kg and a total area of 324 m2. They have a folded volume of 12 m3, which is packed in two separate 6m x 1m x 1m sections. This system provides 47.5 kW of power to recharge the fuel cells. Also, this was designed using the solar radiation flux at Mars. Any time during the mission when we are closer to the sun, we generate more power. This system can also be used to supplement the power from the NTRs in the event of an unusually large power need.</p><p>8.1.5 CTV Power The CTV must provide power only from the time of launch from Mars until docking with the orbiting ERV and from the undocking with the ERV until Earth</p><p>8-1-7 landing. Solar arrays again cannot be used during these stages of the mission. In addition, there is no NTR from which to draw power. RFCs require a large volume of hardware for such a small requirement. Thus, we choose nickel- hydrogen batteries as the power source. The power requirement for the CTV is estimated to be 7 kW. This is based on the capability of the space shuttle to land with only one operating fuel cell1 and the space shuttle fuel cell design of 7 kW.5 A nine-hour discharge time is assumed based on the discharge time designed for the X-38.6 We assume a discharge voltage of 27.5 V with a specific energy of 51.50 W-hr/kg and an energy density of 51.70 W-hr/L.7 A depth of discharge of 0.7 is also assumed. The mass of the battery system is given by</p><p>M=(P * t) / (dd * specific energy) (Eq. 8.1.2) and the volume of the battery system is given by</p><p>V=(P * t) / (dd * energy density) (Eq. 8.1.3)</p><p>With this design, the mass of the battery system is 1800 kg and the volume is 1.74 m3. It provides 7 kW of power to the CTV and can be used as a backup power system on the ERV.</p><p>8.1.6 ERA/ERV Transit Power The ERA draws power from the NTRs while in transit to Mars. Once in orbit around Mars, the ERV deploys an identical set of solar panels to those on the Hab for power. It also has an identical set of RFCs to those on the Hab for periods of eclipse. The solar panels are also the primary power source in transit back to Earth. The RFCs and the batteries on the CTV are backup power sources.</p><p>8.1.7 Conclusions</p><p>8-1-8 The power systems we designed provide ample power throughout the launch, transit, and landing phases of the mission with backup systems in place in the event of a loss in primary power. Future recommendations include refining the solar array structure to ensure support of the array under artificial gravity, further study of the chemistry of the RFCs, and refinement of the RFC system masses. </p><p>8-1-9 8.1.8 References</p><p>1) Space Shuttle, http://www.internationalfuelcells.com/spacedefense/spaceshuttle.shtml, last viewed March 2001</p><p>2) Kohout, Lisa L., “Cryogenic Reactant Storage for Lunar Base Regenerative Fuel Cells,” NASA Lewis Research Center, TM 101980, Cleveland, OH, June 1989</p><p>3) McKissock, Barbara, “Power Systems for Space,” NASA Glenn Research Center, September 2000</p><p>4) TECSTAR, http://www.tecstar.com/basic/main2.htm, last viewed March 2001</p><p>5) ELECTRICAL POWER SYSTEM, http://science.ksc.nasa.gov/shuttle/technology/sts-newsref/sts- eps.html#sts-eps-prsd, last updated August 2000</p><p>6) Baer-Riedhart, Jenny, X-38 Project Summary, http://www.dfrc.nasa.gov/Projects/X38/index.html, last updated February 2001</p><p>7) SPV Batteries, http://www.tech.epcorp.com/pwrsubsys/SPV.htm, last updated August 2000</p><p>8-1-10</p>
Details
-
File Typepdf
-
Upload Time-
-
Content LanguagesEnglish
-
Upload UserAnonymous/Not logged-in
-
File Pages10 Page
-
File Size-