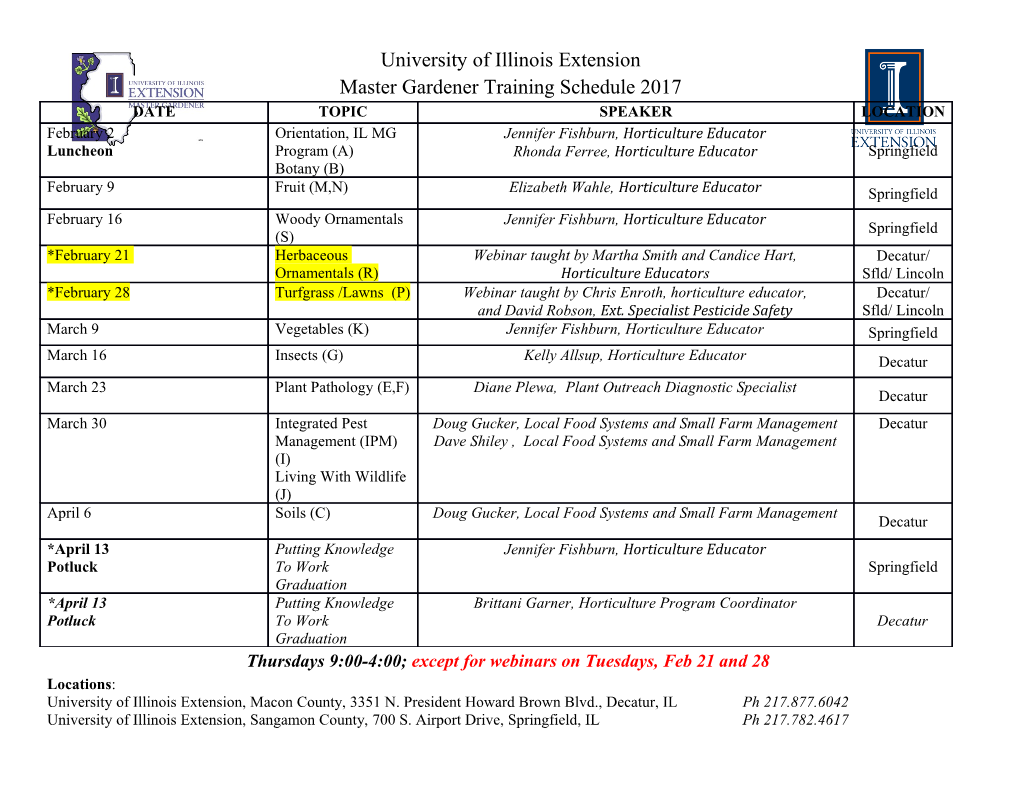
<p>Online Supplementary Materials</p><p>The incidence of low phosphorus soils in Australia</p><p>Robert M Kooyman1,3, Shawn W Laffan2, Mark Westoby1</p><p>1Department of Biological Sciences, Macquarie University, Sydney, New South Wales 2109, Australia; 2Centre for Ecosystem Science, School of Biological, Earth and Environmental Science, University of New South Wales, Sydney, Australia, 2052</p><p>3 Author for communication: Robert Kooyman. Email: [email protected]</p><p>Soil phosphorus studies and methods </p><p>A review of the literature describing studies that measured soil phosphorus (and specifically soil total P) in natural (non-agricultural) areas of native vegetation was undertaken and results were tabulated. A total of 78 studies and 384 locations with quantified values for soil total P were identified. These showed a range of values for soil total P mostly between 10–500 mg kg−1 and within the range identified in the NSSDC. The most commonly used methods for measuring soil total P included butyl-alcohol and hydrogen chloride (hydrochloric acid, HCl) extraction in early studies (e.g. Specht and Rayson 1957), and HCl, colorimetric, x-ray fluorescence (XRF), and inductively coupled plasma optical emission spectrometry (ICP-</p><p>OES) in later studies. In general, where multiple studies at single locations were conducted using different methods through time, we found the results were more or less consistent. </p><p>However, there were exceptions. For example, some studies used ICP-OES after acid digestion and simply reported minimum values as <50 mg kg−1, while others used XRF and reported the minimum detectable limits for soil total P as 10 mg kg−1. The National </p><p>Geochemical Survey of Australia used XRF to measure soil total P (from sediment samples) and reported the continent-wide range as ca 50–2600 mg kg−1 (de Caritat and Cooper 2011).</p><p>An important caveat to the research history quantifying soil P is the considerable variation in methods used, and the acknowledged and ongoing analytical challenges reflected in the complexity of P speciation, P fractionation, and the definition of P pools that include inorganic and organic forms (Chang and Jackson 1957; Sparks et al. 1996; Chen et al. 2015; </p><p>Kruse et al. 2015). In general, P is rock-derived and directly reflects soil parent material. </p><p>Total P is an indicator of non-quartz mineral contributions to soils and is often correlated with other elements (Walker and Syers 1976; Vitousek et al. 2010). </p><p>Seminal ‘early’ research sites</p><p>One set of sites influential in the idea that low nutrient soils favoured sclerophylly was located on Hawkesbury Sandstone near Sydney (Beadle 1962), with total P mostly in the range 30–100 mg kg−1 (woodland/heath and dry sclerophyll forest, Table 2). Another influential set of sites was located on deep podsolised sands in South Australia (Specht and </p><p>Rayson 1957), with total P mostly in the range 10–20 mg kg−1 (Table 2). The particularly influential experiments conducted at Dark Island Heath in South Australia (Specht and </p><p>Rayson 1957) also demonstrated community-level shifts through time in response to field- based fertilizer additions; from sclerophyll heath towards more herbaceous vegetation </p><p>(Specht 1963).</p><p>Low nutrients, drought and sclerophylly</p><p>The discussion about the relative influence of low nutrients versus drought in giving rise to sclerophylly has also been one about the evolutionary history of Australian clades and about what traits should be considered as part of a sclerophylly syndrome (Specht and Rundel 1990;</p><p>Tozer et al. 2015). Through the first half of the Cenozoic (Paleogene to Neogene) Australia is thought to have been under a moist rainforest climate, with seasonal drought appearing ca 20 </p><p>Mya (Oligocene-Miocene). Consequently it has been argued that sclerophyll leaf traits appearing before that must have been adaptations to low nutrients, even if subsequently they proved advantageous as preadaptation to drought (Hill 1998). Scleromorphy in Proteaceae has been evident in Australia from the Late Cretaceous (and suggested as having evolved under wet conditions to enhance water shedding), while xeromorphy was not present until the</p><p>Late Eocene (Hill 1998).</p><p>In early studies, terms such as heathlands (Specht and Rayson 1957), heath, highly sclerophyllous heathlands, shrub-lands, ‘scrub’, and xeromorphic scrub (Beadle 1962) were sometimes used interchangeably. The confounding of ‘xeromorphy’ and ‘sclerophylly’ is discussed in more detail in Hill (1998), and the limitations of defining sclerophylly as a syndrome have been discussed elsewhere (Niinemets 1999; Tozer et al. 2015).</p><p>Leaf traits, sclerophylly, and low-P</p><p>Leaf traits favoured on low-P soils are now reasonably well understood via a series of comparisons between higher versus lower nutrient and higher versus lower rainfall sites </p><p>(Wright and Westoby 1999, 2002, 2003; Wright et al. 2001, 2002, 2003). In that research, species with lower specific leaf area (SLA) tended to achieve longer leaf lifespans (LL) and, at low soil nutrients, species were generally shifted towards especially low SLA – long LL combinations, representing the "slow return" end of the leaf economic spectrum (Wright et al.</p><p>2004). Leaf toughness contributes to leaf longevity by influencing plant-animal interactions such as herbivory, and by reducing the more general causes of leaf injury and loss (Westoby </p><p>1988; Turner 1994; Westoby et al. 2002; Onoda et al. 2011). Investment in tough leaves also influences community and ecosystem functions such as leaf litter decomposition rates and nutrient cycling (Specht and Rayson 1957; Specht 1981; Cornelissen and Thompson 1997; </p><p>Díaz et al. 2004). However, a shift to lower mean SLA at dry sites did not necessarily extend </p><p>LL, seemingly because dry-site species had leaves that were less mechanically robust at a given SLA. This was associated with a tendency for higher leaf N per mass, reflected anatomically in more mesophyll and less lignified tissue – the higher N/mass allowing low rainfall species to economise on water use during photosynthesis (Wright et al. 2003). Of course leaf traits are not the only element in adaptation to low-P soils – there are for example the different root and symbiont strategies responsible for extracting P and filtering species from the available pool along nutrient gradients (Lambers et al. 2008, 2010; Laliberté et al. </p><p>2012; Zemunik et al. 2015). However, leaf traits are the most readily discerned by an observer as corresponding to vegetation with a sclerophyll aspect.</p><p>Old and young landscapes and low and high P</p><p>Old, climatically buffered, infertile landscapes (OCBILs) are described as occupying high points in the landscape and are contrasted with young often disturbed fertile landscapes </p><p>(YODFeLs) that include more recently formed or deposited soils with higher P (Hopper </p><p>2009). Hopper's (2009) contrast between old (OCBIL) versus young (YODFeL) landscapes builds regional processes on top of the local mechanism by which low-P favours long leaf lifespans (see above). </p><p>He postulates that old, nutrient-poor soils in combination with long-term climatic stability </p><p>(exemplified by the inland sandplains of southwest Australia) led to speciation and high regional species richness in sclerophyll floras (Sauquet et al. 2009; Crisp and Cook 2013). </p><p>However this world-view has been challenged on several fronts. For example, a recent re- evaluation of geomorphological evidence for the southwest of Australia indicates significant reworking of the inland sandplains during the Quaternary and limited climatic buffering largely constrained to coastal areas (Wyrwoll et al. 2014). It has also been suggested that during the Pleistocene, and somewhat similar to the rainforests in Australia (Kooyman et al. 2013), sclerophyll floras contracted and expanded as a consequence of glacial-interglacial climate cycles that increased aridity and the incidence of fire (Sniderman and Haberle 2012). </p><p>Interestingly, the fossil record confirms that hyper-diverse sclerophyll floras with small- leaved species in heath lineages were more widespread and present in high rainfall summer- wet (non-mediterranean climate) regions of the continent from at least the early Pleistocene </p><p>(Sniderman et al. 2013). The fossil record also shows that sclerophyll leaves from wet environments were present in the Paleogene (Hill 1998; Hill and Brodribb 2001; Jordan et al. </p><p>2005), long before the onset of aridity.</p><p>National Soil Site Data Collation (NSSDC) overview</p><p>Continental distribution of soil sites with total P values is shown in Fig. S1, range relative to longitude in Fig. S2, and distribution of very low P values in Fig. S3. The NSSDC includes the Australian Soil Resource Information System data for soil samples (ASRIS; Johnston et al. 2003). We present the results using means (for soil total P) by unique location. We tested the influence of variation in the 0-30cm depth layer by analysing the minimum values (Table </p><p>S1). While the percentage of locations with low values increased (as would be expected) in all regions, the overall relative (regional) frequency distribution patterns remained.</p><p>Table S1 Cumulative percentage soil total P values based on minimums by location.</p><p>MINIMUMS Cumulative % soil total P values</p><p>Sample_Region Nos. 10 mg kg−1 ≤20 mg kg−1 ≤50 mg kg−1 ≤100 mg kg−1 ≤250 mg kg−1 ≤500 mg kg−1 NSSDC area-weighted 9234 1.04 3.18 14.4 33.32 65.12 88.46 NSSDC unweighted 9234 1.34 3.18 11.97 29.23 59.24 82.81 SW Western Australia 1200 4 10.83 35.45 64.77 92.43 97.53 Eastern Australia 5859 0.29 0.82 5.68 23.81 56.03 80.63 Southeast South Australia 402 1.6 10.83 22.85 43.98 79.67 95.63 Central Deserts 314 - - 4.2 21.83 69.62 96.75 Western Monsoon Tropics 474 0.92 4.97 40.67 71.17 92.3 98.94 The selection of focal regions for comparison was designed to achieve continental coverage but was limited by the distribution of continental site samples in the NSSDC data set (Fig. 1; </p><p>Figs S1-S3) including in relation to the classic ecological study locations (Specht and Rayson</p><p>1957; Beadle 1962). 5 1 - 0 2 - 5 2 - e d u t i t a L 0 3 - 5 3 - 0 4 -</p><p>120 130 140 150</p><p>Longitude</p><p>Fig. S1 Distribution of 9234 unique NSSDC samples of soil total phosphorus from the 0-</p><p>30cm depth category. Note the paucity of sampling in many regions including parts of </p><p>Central and Western Australia, New South Wales, Victoria and western Tasmania. 0 0 2 . 0 0 0 1 . 0 0 m 5 c 0 0 . 3 0 - 0 _ % 0 _ 2 s 0 u . r 0 o h p 0 s 1 o 0 . h 0 p _ l a 5 t 0 o t 0 . 0 2 0 0 . 0 1 0 0 . 0</p><p>120 130 140 150</p><p>Longitude</p><p>Fig. S2 Distribution of 9234 unique NSSDC samples of soil total phosphorus values (%) from the 0–30cm depth category relative to Longitude (west to east). y-axis log-scaled. Units convert as 0.001 % P equals 10 mg kg−1 P. 5 1 - 0 2 - 5 2 - e d u t i t a L 0 3 - 5 3 - 0 4 -</p><p>120 130 140 150</p><p>Longitude</p><p>Fig. S3 Distribution of 758 unique NSSDC samples of soil total phosphorus values ≤50 mg kg−1 from the 0–30cm depth category.</p><p>Other data sources: Soil and Landscape Grid of Australia (SLGA)</p><p>The Soil and Landscape Grid of Australia (SLGA; Viscarra Rossell et al. 2015; Grundy et al. </p><p>2015; http://www.clw.csiro.au/aclep/soilandlandscapegrid/) provides access to data layers for modelled soil attributes (including soil total P) at 3 arc second (90 x 90m) intervals. Maps provide soil total P values colour coded in the key from <200 mg kg−1 (0.02 %) to >500 mg kg−1 (>0.05 %); while the data sets of modelled P values (downloaded from GIS) show estimated values from 50 mg kg−1 (0.005 %) to >1000 mg kg−1 (0.1 %). http://www.clw.csiro.au/aclep/soilandlandscapegrid/ProductDetails-SoilAttributes.html</p><p>Data Limitations in relation to SLGA</p><p>We investigated the use of the SLGA geospatial layers for this research. However, the modelling approach appears to have caused a contraction of the left tail of the distribution </p><p>(e.g., Fig. S4 upper), and it is this tail that is the focus of this research. Our initial efforts to generate area-weighted frequency distributions for soil total P values based on the modelled </p><p>SLGA map data layers were constrained by several factors. First, it may be the case that very low P values were not included in the model. Second, the CUBIST model (that generated the </p><p>SLGA map estimates) used multiple linear regressions (OLS) of variables ordered by a decision tree (Viscarra Rossell and Bui 2015). A predictable consequence of using OLS regression was a narrowing of the range of variation in soil total P estimates toward the mean.</p><p>This contraction has the effect that the percentage of the distribution lying in the low-P tail is considerably smaller in the SLGA estimates (e.g. Fig. S4 upper) than is known to be the case in reality (Fig. S4 lower). Our focus accordingly shifted to the measured data from soil site samples provided in the NSSDC data set.</p><p>SLGA modelling methods in more detail</p><p>The SLGA estimates are generated using a two-component spatial modelling process allied with bootstrap resampling (Viscarra Rossell and Bui 2015). The first component of the spatial modelling uses the CUBIST software, which combines a hierarchical decision tree process with multiple linear regressions (OLS) such that the individual regressions are applied to less complex subsets of the data. In essence the approach avoids the need to represent the full system in one model with all its associated complexity. As with any regression approach, there will be a contraction of the range of variation in soil total P estimated values toward the mean. To reinstate some of this variation in the SLGA, the spatial distribution of CUBIST model residuals were interpolated using kriging and then added to the CUBIST estimates, a process analogous to Universal Kriging. The intent is to reinstate much of the variation, but spatial interpolation is a smoothing process so the more ‘extreme’ values (both high and low, and including those of the left tail) are unlikely to be modelled and thus the final estimates remain unlikely to contain these values (e.g., Fig. S4 upper). The resultant contraction is broadly consistent with the reported r2 scores of 0.45 for total P for the SLGA depth intervals spanning 0-30 cm (Viscarra Rossell and Bui 2015, Table 1). Fig. S4 Upper: Frequency distribution (cumulative % scaled from 0–1) of SLGA 3 arc second estimated values as data points for log-scaled soil total P as percent concentration </p><p>(Log_Phosphorus %) values in Swan Coastal Plain region (n = 2049989 data points). A phosphorus % of 0.01 corresponds to 100 mg kg−1. Lower: NSSDC data (un-weighted) for the Swan Plain showing actual measured spread of soil total P values from soil sample sites. </p><p>Note narrower range of variation in the upper graph. We believe this arises because OLS regression is central to the estimating process used in the SLGA ("regression to the mean"). It may also be the case that low P values were not included. Possibly spatial smoothing also contributes to the narrowing of the range of variation. References</p><p>Chang SC, Jackson ML (1957) Fractionation of soil phosphorus. Soil Sci 84:133–144</p><p>Chen CR, Hou EQ, Condron LM, Bacon G, Esfandbod M, Olley J, Turner BL (2015) Soil phosphorus fractionation and nutrient dynamics along the Cooloola coastal dune chronosequence, southern Queensland, Australia. Geoderma 257-258:4–13</p><p>Cornelissen JHC, Thompson K (1997) Functional leaf attributes predict litter decomposition rate in herbaceous plants. New Phytol 135:109–114</p><p>Crisp MD, Cook LG (2013) How was the Australian flora assembled over the last 65 million years? A molecular phylogenetic perspective. Annu Rev Ecol Syst 44:303–324</p><p> de Caritat P, Cooper M (2011) National Geochemical Survey of Australia: The </p><p>Geochemical Atlas of Australia: Dataset. Geoscience Australia, Canberra. http://dx.doi.org/10.4225/25/54CAB00B4C9AB</p><p>Díaz S, Hodgson JG, Thompson K, Cabido M, Cornelissen JHC, Jalili A, et al (2004) The plant traits that drive ecosystems: evidence from three continents. J Veg Sci 15:295–304</p><p>Grundy MJ, Viscarra Rossel RA, Searle RD, Wilson PL, Chen C, Gregory LJ (2015) The soil and landscape grid of Australia. Soil Res 53:835–844 Hill RS (1998) Fossil evidence for the onset of xeromorphy and scleromorphy in Australian </p><p>Proteaceae. Aust Syst Bot 11:391–400</p><p>Hill RS and Brodribb TJ (2001) Macrofossil evidence for the onset of xeromorphy in </p><p>Australian Casuarrinaceae and tribe Banksieae (Proteaceae). J Mediterr Ecol 2:127–136</p><p>Jones R, Groves RH, Specht RL (1969) Growth of heath vegetation III. Growth curves for heaths in southern Australia: a reassessment. Aust J Bot 17:309–314</p><p>Jordan GJ, Dillon RA, Weston PH (2005) Solar radiation as a factor in the evolution of scleromorphic leaf anatomy in Proteaceae. Am J Bot 92:789–796</p><p>Kooyman RM, Rossetto M, Sauquet H, Laffan SW (2013) Landscape patterns in rainforest phylogenetic signal: isolated islands of refugia or structured continental distributions? PLoS </p><p>ONE 8(12): e80685. doi:10.1371/journal.pone.0080685</p><p>Kruse J, Abraham M, Amelung W, Baum C, Bol R, Kühn O, Lewandowski H, Niederberger </p><p>J, Oelmann Y, Rüger C, Santner J, Siebers M, Siebers N, Spohn M, Vestergren J, Vogts A, </p><p>Leinweber P (2015) Innovative methods in soil phosphorus research: A review. J Plant Nutr </p><p>Soil Sci 178:43–88</p><p>Laliberté E, Turner BL, Costes T, Pearse SJ, Wyrwoll K-H, Zemunik G, Lambers H (2012) </p><p>Experimental assessment of nutrient limitation along a 2-million-year dune chronosequence in the south-western Australia biodiversity hotspot. J Ecol 100:631–642 Lambers H, Raven JA, Shaver GR, Smith SE (2008) Plant nutrient-acquisition strategies change with soil age. Trends Ecol Evol 23:95–103</p><p>Lambers H, Brundrett MC, Raven JA, Hopper SD (2010) Plant mineral nutrition in ancient landscapes: high plant species diversity on infertile soils is linked to functional diversity for nutritional strategies. Plant Soil 334:11-31</p><p>Niinemets Ü (1999) Research review. Components of leaf dry mass per area—thickness and density—alter leaf photosynthetic capacity in reverse directions in woody plants. New Phytol</p><p>144:35–47</p><p>Onoda Y, Westoby M, Adler PB, Choong AMF, Fiona J. Clissold FJ, Cornelissen JHC, Díaz </p><p>S, Dominy NJ, Elgart A, Enrico L, Fine PVA, Howard JJ, Jalili A, Kitajima K, Kurokawa H, </p><p>McArthur C, Lucas PW, Markesteijn L, Pérez-Harguindeguy N, Poorter L, Richards L, </p><p>Santiago LS, Sosinski Jr EE, Van Bael SA, Warton DI, Wright IJ, Yamashita N (2011) </p><p>Global patterns of leaf mechanical properties. Ecol Letts 14:301–312</p><p>Sauquet H, Weston PH, Anderson CL, Barker NP, Cantrill DJ, Mast AR, Savolainen V </p><p>(2009) Contrasted patterns of hyperdiversification in Mediterranean hotspots. Proc Natl Acad</p><p>Sci USA 106:221–225</p><p>Sniderman JMK, Haberle SG (2012) Fire and vegetation change during the Early Pleistocene in southeastern Australia. J Quaternary Sci 27:307–317 Sniderman JMK, Jordan GJ, Cowling RM (2013) Fossil evidence for a hyperdiverse sclerophyll flora under a non-Mediterranean-type climate. Proc Natl Acad Sci USA </p><p>110:3423–3428</p><p>Sparks DL, Page AL, Helmke PA, Loeppert RH (1996) Methods of Soil analysis, Part 3, </p><p>Chemical Methods. Soil Science Society of America Inc., Madison, WI, USA</p><p>Specht RL (1963) Dark Island heath (Ninety-Mile Plain, South Australia) VII The effect of fertilizers on composition and growth, 1950-1960. Aust J Bot 11:67–94</p><p>Specht RL (1981) Nutrient release from decomposing leaf litter of Banksia ornata, Dark </p><p>Island heathland, South Australia. Aust J Ecol 6:59–63</p><p>Specht RL, Rayson P (1957) Dark Island heath (Ninety-Mile Plain, South Australia) I </p><p>Definition of the ecosystem. Aust J Bot 5:52–85</p><p>Specht RL, Rayson P, Jackman ME (1958) Dark Island heath (Ninety-Mile Plain, South </p><p>Australia) VI Pyric succession: changes in composition, coverage, dry weight, and mineral nutrient status. Aust J Bot 6:59–88</p><p>Specht RL, Rundel PW (1990) Sclerophylly and foliar nutrient status of mediterranean- climate plant communities in southern Australia. Aust J Bot 38:459–474</p><p>Tozer WC, Rice B, Westoby M (2015) Evolutionary divergence of leaf width and its correlates. Am J Bot 102:367–378 Turner IM (1994) Sclerophylly: Primarily protective? Funct Ecol 8:669–675</p><p>Viscarra Rossel RA, Bui EN (2015) A new detailed map of total phosphorus stocks in </p><p>Australian soil. Science of the Total Environment http://dx.doi.org/10.1016/j.scitotenv.2015.09.119</p><p>Viscarra Rossel RA, Chen C, Grundy MJ, Searle R, Clifford D, Campbell PH (2015) The </p><p>Australian three-dimensional soil grid: Australia’s contribution to the GlobalSoilMap project.</p><p>Soil Research http://dx.doi.org/10.1071/SR14366</p><p>Vitousek PM, Porder S, Houlton BZ, Chadwick OA (2010) Terrestrial phosphorus limitation: mechanisms, implications, and nitrogen–phosphorus interactions. Ecol Appl 20:5–15</p><p>Walker TW, Syers JK (1976) The fate of phosphorus during pedogenesis. Geoderma 15:1–19</p><p>Westoby M (1988) Comparing Australian ecosystems to those elsewhere. BioScience </p><p>38:549–556</p><p>Westoby M, Falster DS, Moles AT, Vesk PA, Wright IJ (2002) Plant ecological strategies: some leading dimensions of variation between species (Review). Annu Rev Ecol Syst </p><p>33:125–159 Wright IJ, Westoby M (1999) Differences in seedling growth behaviour among species: trait correlations across species, and trait shifts along nutrient compared to rainfall gradients. J </p><p>Ecol 87:85–97</p><p>Wright IJ, Westoby M (2002) Leaves at low versus high rainfall: coordination of structure, lifespan and physiology. New Phytol 155:403–416</p><p>Wright IJ, Westoby M (2003) Nutrient concentration, resorption and lifespan: leaf traits of </p><p>Australian sclerophyll species. Funct Ecol 17:10–19</p><p>Wright IJ, Westoby M, Reich PB (2002) Convergence towards higher leaf mass per area in dry and nutrient-poor habitats has different consequences for leaf life span. J Ecol 90:534–</p><p>543</p><p>Wright IJ, Reich PB, Westoby M (2003) Least-cost input mixtures of water and nitrogen for photosynthesis. Am Nat 161:98–111</p><p>Wright IJ, Reich PB, Westoby M (2001) Strategy shifts in leaf physiology, structure and nutrient content between species of high- and low-rainfall and high- and low-nutrient habitats. Funct Ecol 15:423–434</p><p>Wright IJ, Reich PB, Westoby M, Ackerly DD, Baruch Z, Bongers F, et al (2004) The worldwide leaf economics spectrum. Nature 428:821–827 Wyrwoll K-H, Turner BL, Findlater P (2014) On the origins, geomorphology and soils of the sandplains of south-western Australia. In: Lambers H (ed) Plant life on the sandplains in southwest Australia: a global biodiversity hotspot, University of Western Australia </p><p>Publishing, pp 3–21</p><p>Zemunik G, Turner BL, Lambers H, Laliberté E (2015) Diversity of plant nutrient-acquisition strategies increases during long-term ecosystem development. Nature Plants DOI: </p><p>10.1038/NPLANTS.2015.50</p>
Details
-
File Typepdf
-
Upload Time-
-
Content LanguagesEnglish
-
Upload UserAnonymous/Not logged-in
-
File Pages18 Page
-
File Size-