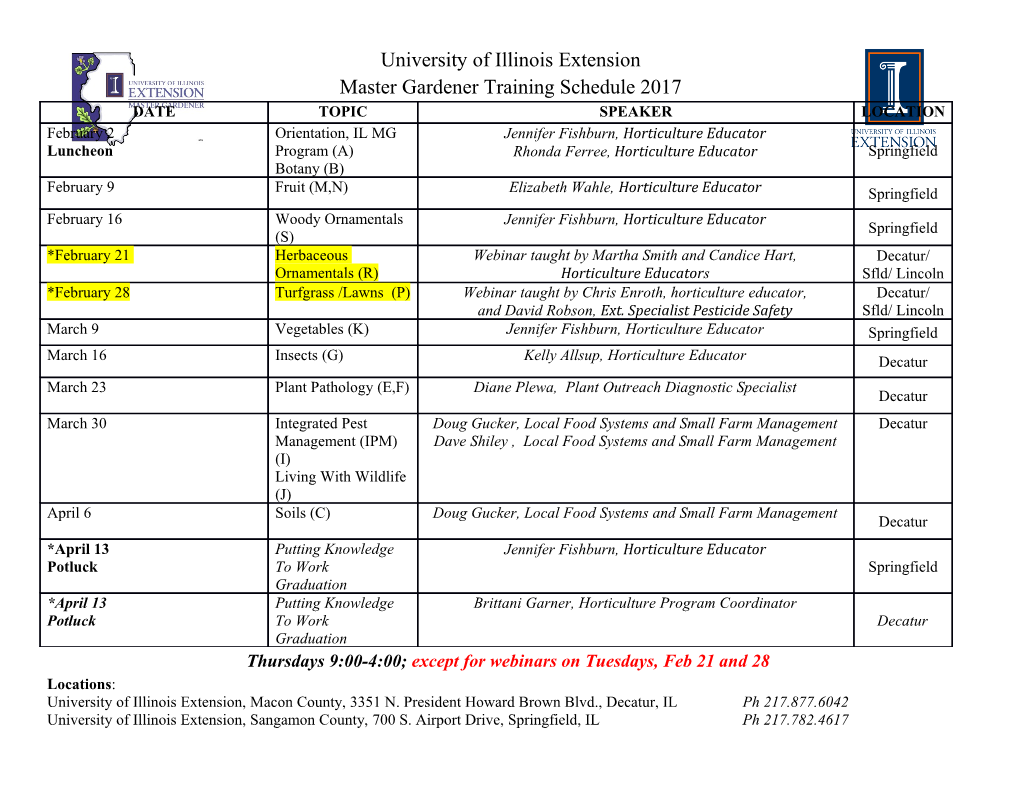
<p> β phase decomposition in Ti-5Al-5Mo-5V-3Cr</p><p>N. G. Jones1, R.J. Dashwood2, M. Jackson3, D. Dye1 1. Department of Materials, Imperial College London, London, SW7 2AZ 2. Warwick Engineering Group, University of Warwick, Coventry, CV4 7AL 3. Department of Engineering Materials, University of Sheffield, Sheffield, S1 3JD </p><p>Keywords: Titanium alloys, phase transformations, TEM, synchrotron radiation</p><p>Abstract: The decomposition of the β phase during rapid cooling of the near β titanium alloy Ti-5Al-5Mo-5V- 3Cr has been studied using in-situ X-ray synchrotron diffraction combined with ex-situ conventional laboratory X-ray diffraction and transmission electron microscopy. Evidence is found supporting the suggestion by De Fontaine et al that embryonic ω structures form by the correlation of linear (111)β defects at high temperatures. Further cooling causes increased correlation of these defects and the formation of athermal ω structures within the β matrix at temperatures ~500°C. Post-quench aging at 570°C resulted in the nucleation of α laths after ~90 seconds at temperature, with the laths all initially belonging to a single variant type. Aging for 30 minutes produced an even distribution of α precipitates with a lath morphology approx. 1.5 by 0.2 µm in size composed of both the expected Burgers variants. Mechanical property data suggests that the ω structures alone have no real effect, however hardness increases were observed as the α phase developed. The utilisation of thermal regimes similar to those presented in this paper could offer a method to engineer the α phase in near β titanium alloys and hence control mechanical properties. Introduction</p><p>Ti-5Al-5Mo-5V-3Cr (Ti-5-5-5-3) is a relatively new near beta alloy based on the Russian alloy VT22. This high strength alloy is of particular interest to the aerospace industry for applications such as bogie beams in aircraft landing gear. Economic manufacturing of these large components is achieved by hot near net shape forging [1], which succeeds in obtaining the desired mechanical properties. However, many near beta alloys are extremely sensitive to forming variables, even during isothermal processing. For example, Ti-10V-2Fe-3Al (Ti-10-2-3), which is used in landing gear forgings for the Boeing 777, has been shown to be particularly sensitive to small fluctuations in both temperature and strain rate [2]. Ti-5-5-5-3 meanwhile, has exhibited less sensitivity to forming variables and offers a larger processing window due to the less steep beta transus approach curve; hence it is becoming the alloy of choice for airframe forgings [3, 4].</p><p>One of the major reasons near beta alloys are selected for such applications is their ability to achieve strengths well over 1 GPa [5]. One explanation as to the source of this high strength is the development of fine scale α precipitates (~20 nm by 200 nm in Ti-10-2-3 [6-8]). These precipitates create a high number of α/β interfaces that act as dislocation barriers, increasing the alloy strength [6]. Producing an evenly distributed fine scale α structure requires multiple site nucleation and limited growth. Sympathetic nucleation from primary α or nucleation of α from martensitic (α’’) plates would require the larger structural units to already be present in the microstructure. Therefore to achieve the desired fine precipitates, nucleation would need to occur from dislocations, grain boundaries, vacancies and/or another phase, such as the ω phase. </p><p>Ti-5-5-5-3 has been shown to vary from VT22 in that when quenched from ~ 50°C above its beta transus a 100% retained β structure is not achieved. Several researchers have reported the formation of athermal ω when Ti-5-5-5-3 is rapidly cooled from the β phase field [3, 9, 10]. The formation mechanism of the compositionally invariant athermal ω, by the collapse of β {111} planes, has been well documented [6, 11, 12]. The resulting precipitates are usually found to be small (~ 2 – 10 nm) ellipsoidal structures aligned along <111>β [12]. </p><p>It has been suggested that at high temperatures, well above the supposed ω start temperature (300 - 500°C in near β alloys [6]), embryonic ω or “ω-like fluctuations” are present [11, 13, 14]. These structures can be thought of as a bcc lattice defect produced by atomic jumps in the <111> direction causing local plane collapse. The highly correlated displacements give significant concentrations, even at high temperatures, where the structure is maintained for longer than a typical atomic vibration [12]. At higher temperatures the embryos persist as uncorrelated line defects aligned with the <111> direction, meaning that each diffracts differently until cooled closer to the ω transition temperature, when the correlation increases and discrete ω particles are formed [12]. </p><p>The development of the athermal ω has been studied using high resolution transmission electron microscopy (HRTEM) [15]. It has been suggested that as alloy solute concentration increases the athermal ω structure becomes incommensurate, leading to the commonly observed diffuse streaking in electron diffraction patterns [15]. Lattice imaging has shown a deviation of the apparent ω c-axis away from the <111> β, rotating by up to 10° towards the [110]. This has been linked to the nature of the stepped interface between the ω and β formed by the coalescence of multiple ω embryos. The local selection of the collapsing plane for a given ω structures is random and for each individual case will be aligned with the <111>β. When two structures are joined using a single vector a deviation from the <111>β can arise [15]. </p><p>Due to the small size of athermal ω many researchers have subsequently aged samples at low temperatures (~ 300 – 400°C), often below α formation temperatures, in order to allow growth of the ω phase so that characterisation becomes simpler. However, within this regime, diffusion occurs and solute segregation in the <100> direction gives rise to the incoherent and compositionally variant isothermal ω [12]. The isothermal ω phase has been reported as having both cuboidal and ellipsoidal morphologies, the development of which is governed by the misfit in the alloy system [6], and a larger size (10 2 0 nm) than that of athermal ω. </p><p>Small volume fractions of athermal ω appear to have no significant effect on mechanical properties, but there has been interest in their role on subsequent phase transformations [6]. The ability of ω particles to act as nucleation sites for α has been established, however the exact nature of the interaction is still unclear. The orientation relationship between β α is very well documented; (110)β // (0001)α with [111]β // [11 2 0]α [16]. The β ω relationship has been defined as (111)β // (0001)ω with [1 1 0]β // [11 2 0]ω [11, 17]. Using a similar approach, the orientation relationship between the α and ω can be hypothesized to be (11 2 0)ω // (0001)α with [0001]ω // [11 2 0]α. Ohmori et al [8] used HRTEM to image the direct nucleation of an α lath on the interface between β and isothermal ω. The observations suggested that a (10 1 0)α // (1 1 00)ω // (211)β orientation relationship occurred, which is consistent with the Burgers relationship between β and α.</p><p>Recent publications concerning the aging of various β titanium alloys have found that the rate of heating to peak aging temperature can affect the size and distribution of the α phase [18]. It is hypothesised that slow heating (0.25°Cmin-1) from room temperature to α aging temperature allows small isothermal ω precipitates to nucleate from retained β microstructure. These subsequently act as nucleation sites for α during the aging process and give rise to a well distributed fine lath α structure. At faster heating rates (20°Cmin-1), ω is not observed and the resultant α structure is less well distributed and exists as thick laths. From this evidence, the authors conclude that the speed at which components are heated to final aging temperature has a significant effect on the final mechanical properties.</p><p>Clearly the development of the α phase during processing can have a dramatic effect on the final mechanical properties. The applications that near beta alloys are utilised for, such as landing gear, require high strength, high fracture toughness and good fatigue properties. Deliberate low temperature (ω) aging producing fine scale α gives rise to high strength, low work hardening, lower ductility and lower fracture toughness when compared to α aged material [6]. Fatigue crack growth is slower in ω aged material than α aged, however, the effect on crack initiation has not been well reported and α aged smooth bar samples have been shown to have longer fatigue life times than corresponding ω aged bars [19].</p><p>The possibility of engineering the α phase in near beta alloys to achieve improved properties and microstructure control is tantalising and therefore of interest for investigation. If the size, morphology, density and volume fraction of the reinforcing phase can be controlled, then tailoring the microstructure to specific design requirements could become possible. Combining the high strength characteristics of multiple site nucleated ω aged material with the ductility, fracture toughness and fatigue properties of the α aged material would be ideal. A possible method for achieving such a microstructure would be to age material, already containing ω particles, in the α phase region.</p><p>Before such microstructural tailoring can be developed, a better understanding of the processes involved is required, and as such this paper characterises the β ω athermal α transformations in Ti-5-5-5-3, utilising in-situ X-ray synchrotron experiments, and ex-situ transmission electron microscopy. The use of a short wavelength, highly penetrating monochromated X-ray beam from a synchrotron source allows diffraction patterns to be obtained with both high d-spacing resolution and time resolution of better than 1s. This allows insight into the possible mechanisms of microstructural development to be obtained.</p><p>Experimental</p><p>A cylindrical billet (67mm diameter by 79mm) of Ti-5-5-5-3, with a β transus temperature of ~845°C [3] was solution heat treated at 980°C for 60 minutes. The composition is given in Table 1. The hot billet was transferred to an ENEFCO 5 MN press and extruded to round bar form using a reduction ratio of 10:1. All samples used in the following studies were machined from this bar stock.</p><p>In-situ experiments In-situ experiments were conducted at the ID15b beamline of the European Synchrotron Radiation Facility (ESRF) using high penetration X-rays of wavelength λ = 0.1415 Ǻ to enable high resolution diffraction data to be obtained in transmission. Small bar samples, 1mm x 1.75mm x 36mm, were held in the monochromated beam path using an Instron Electro-Thermal Mechanical Testing machine (ETMT). Diffraction data was collected by a 2D PIXIUM 4700 area X-ray detector at a distance of 647.5 mm and stored for later analysis using ESRF software Fit 2D1.</p><p>Samples were heated into the β phase field (950°C) in an argon filled enclosure using resistance heating. Temperature was monitored and controlled via an R type thermocouple spot welded to the sample surface, directly above the beam position. Samples were homogenised in the β phase field before being cooled by conduction to room temperature. Quench cooling rates (~ 1800°C min - 1) were obtained. The samples were then heated to 570°C, within the industrial α aging range for the alloy [5], at a rate of ~ 950°C min-1 in order to avoid the formation of isothermal ω. Samples were aged for a total of 15 minutes, allowing the observation of initial α phase nucleation and growth.</p><p>Whilst in-situ experiments provide an excellent opportunity to witness events in real time as they occur, the ability to utilise other methods of characterisation is lost. Therefore an ex-situ study was also employed, designed to mirror the procedures from the in-situ experiments as closely as possible, to enable further interrupted testing and investigation of the transformation behaviour.</p><p>Ex-situ experiments A 20mm diameter by 100mm height bar was solution heat treated at 900°C for 30 minutes in an infrared furnace2 before being water quenched. Small disc-like samples, 1.5mm in thickness, were subsequently machined using a Struers Accutom 5 before being aged.</p><p>Disc samples were suspended in the middle of the infrared furnace from a K type thermocouple spot welded to the sample surface. Heating to the aging temperature of 570°C was carried at 300°Cmin-1, again sufficient to bypass the isothermal ω forming region, before being subjected to a water quench after the following aging times; 1, 3, 5, 10, 15 and 30 minutes.</p><p>Aged samples were characterised by conventional X-ray diffraction on a Philips PW 1710 diffractometer (Copper K weighted average λ = 1.5418 Å) in the angular (2θ) range of 30 to 90 and Vickers hardness values obtained using a 10kN load. Transmission Electron Microscopy (TEM) samples were prepared by even grinding of sections of each disc to a thickness of 0.2mm before</p><p>1 Fit 2D, V.12.077 A. Hammersley (ESRF) 2005 2 Severn Furnaces 1000°C radiant furnace controlled using K-type thermocouple connected to a Eurotherm 902P controller final thinning by electropolishing using 10%H2SO4 in CH3OH at -40°C. Thin foils were inspected using a Jeol 2000FX II Transmission Electron Microscope. </p><p>Results and Discussion</p><p>The phase transformations occurring in Ti-5-5-5-3 during rapid cooling from the β phase field were studied using in-situ X-ray synchrotron diffraction; the experimental time-temperature scheme and selected diffraction patterns are shown in Figure 1. The full ring diffraction pattern corresponding to the β phase achieved following a 600 s solution heat treatment at 950°C is shown in Figure 1a. After rapid cooling to room temperature secondary peaks are observed in the diffraction pattern, corresponding to the ω phase (Figure 1b). Heating this structure to an aging temperature of 570°C at a rate of ~950°Cmin-1 resulted in a decrease in the intensity of the ω peaks (Figure 1c). Aging for 900s at 570°C resulted in the loss of the ω phase and the appearance of peaks corresponding to the hexagonal α phase (Figure 1d). </p><p>The formation of athermal ω during rapid cooling is examined in Figure 2, which shows synchrotron diffraction data around the β (110) peak at two second intervals during rapid cooling from the β phase field. At 950°C the material is ~100°C above its β transus and a single distinct peak corresponding to the β(110) was observed. Soon after commencing the rapid cooling a shoulder to the left hand side of the main β (110) peak appeared which becomes a distinct peak between 624°C and 470°C. This observation is consistent with the formation of embryonic ω as discussed in the introduction. High in the β phase field (950°C) uncorrelated linear (111)β defects exist but diffract independently and therefore a single distinct β peak is observed. During rapid cooling the defects become more correlated, forming ω embryos which diffract to produce the peak shoulder seen at 885°C and 624°C. It is inferred that the ω start line is crossed during continued rapid cooling, by the appearance of a second peak in the place of the shoulders at 470°C. The presence of ω particles at this temperature is consistent with reported ω start temperatures (~500°C) for similar alloy systems [6].</p><p>The time scales corresponding to α formation during aging can also be evaluated using data around the β (110) peak, as both the 100% peaks for ω and α phases occur in this region. Synchrotron diffraction from aging an as-quenched sample of Ti-5-5-5-3 at 570°C data is shown at 30 second intervals in Figure 3. The first observation of the α phase occurs after 120 seconds, manifested as a small perturbation to the right of the main β peak corresponding to the α (10 1 1). Peak intensity increases as the aging proceeds and more α nucleates and grows. After 300 seconds of aging at 570°C appearance of the α (0002) peak was observed to the left of the β (110). </p><p>During the initial stages of aging the ω (10 1 1) peak, present following the rapid cooling, decreases in intensity, becoming less discrete and finally being indistinguishable after 210 seconds. </p><p>Data from the ex-situ study support the findings of the in-situ experiment; conventional X-ray diffraction data for samples can be seen in Figure 4. The greater energy and angular divergence utilised compared to the synchrotron experimentation does not allow the ω (10 1 1) reflection to be resolved, however, the nucleation of the α phase is detected after 5 minutes aging. The slight time difference between the two studies before distinct α peaks become apparent can be accounted for by the time spent at the aging temperature due to different heating rates (~ 90s for in-situ and between 70 and 190s for ex-situ) and the higher resolution of the synchrotron measurements.</p><p>The presence of the ω phase following water quenching can clearly be identified from electron diffraction in the [110]β zone axis with the typical diffuse streaked ‘x’ pattern and intense ω maxima between β reflections present in Figure 5a. There are four intensity maxima corresponding to the [10 1 1], [ 1 010] and [ 1 011] lying on the diffuse streaks which do not lie in the commensurate positions of 1/3 and 2/3 [112]β. Instead the reflections from the two variants, [10 1 1] / [ 1 010] and [ 1 011] / [ 1 010], are shifted towards each other. This has been observed in other Ti alloys and has been rationalised by De Fontaine [17]. It was suggested that close to the ω transition temperature, when the collapsed {111} planes are only locally correlated, the strain fields of different variants interact. This interaction effectively couples the variants, and as coupling increases the streaks adopt a more circular form, corresponding to the octahedral site, Figure 5c. </p><p>Sinkler and Luzzi [20] have reported a similar behaviour of ω reflections whilst investigating Ti-Cr alloys. They suggested instead that the shifts in position were due to variations in the ratio of collapsed to normal {111}β planes in the local ω structure and to chemical ordering. By inserting extra pairs of collapsed planes the ω structure can expanded from the expected ω (3) to ω(5) or ω(7), causing reflections to move towards each other along the [111]β. It was also noted that the stronger β stabilising elements (Fe, Cr and Mo) cause a greater shift in position of the ω reflections than weaker stabilising elements (Nb and V). This was thought to stem from short range chemical ordering which when decreased returned the ω reflections to the expected Bragg positions. </p><p>In a Ti-15Mo alloy the diffuse streaking was initially observed, close to the ω transformation temperature, to form in a circular pattern and became progressively more linear as the temperature decreased [17]. This was attributed to a change from short range order to long range order and therefore to a reduction in the coupling effect. In the current study neither distinct reflections nor streaking were rectilinear at temperatures far below the ω start temperature. The increased solute content (~23 at. %) of Ti-5-5-5-3, when compared to Ti-15Mo, would be expected to increase the strain field coupling effect [17]. This would therefore suggest that the ordering in the system remains short range and the ω structures very small, consistent with the weak reflections observed in Figure 5.</p><p>From a theoretical standpoint the ( 2 021)ω and (30 3 0)ω reflections would be obscured by the (200)β and (211)β reflections respectively and in addition the coupling effects above can make determining the ω lattice parameters difficult. However, distinct reflections can be seen in Figure 5b outside the β matrix reflections. Using Figure 5b a β lattice parameter of 3.21 Å was obtained which exhibits close correspondence to the 3.22 Å obtained from the major β peak in Figure 2 when fitted using a Voigt function. From the β lattice parameter theoretical lattice parameters for ω can be calculated, aω= 4.55 Å and cω=2.79 Å, from well established geometric assumptions, aw = 2ab and cw = 3 2 ab [11]. Using the ( 2 021)ω and (30 3 0)ω reflections from Figure 5b, experimental lattice parameters can be calculated, aω= 4.37 Å and cω=2.65 Å, which show good correlation with the theoretical values.</p><p>It is interesting to note that the (10 1 1)ω interplanar spacing derived from the in-situ X-ray diffraction varies when compared to that derived from the ex-situ electron diffraction patterns. The spacing between (10 1 1)ω planes is larger in the ex-situ study by ~0.3 Å. This variation is thought to be related to the cooling rate and suggests that the local ω structure is not identical in the two studies. Previous research investigating isothermal ω report electron diffraction patterns with ω reflections at the commensurate positions with no diffuse streaking. From these studies it can be concluded that when ω is isothermally precipitated by low temperature aging there is no variation from the ω (3) structure. However, when produced via rapid cooling variations to both structure and orientation occur due to the random nature of the local plane collapse, for example the ω(5) and ω(7) structures [20].</p><p>While a rapid cooling rate was achieved during the in-situ experiments it is anticipated that the water quenching employed in the ex-situ study provided faster cooling. Sinkler and Luzzi [20], show that as the ratio of collapsed to un-collapsed planes pairs within the ω structure increases the distance, in reciprocal space, between (0001) and (0002) planes decreases. Thus the larger (10- 11)ω inter planar spacing observed following a faster cooling rate would suggest a larger average ω structural unit size, which would be reduced by slower cooling.</p><p>Aging for 5 minutes resulted in the precipitation of small, ~150 nm in length by 10 nm wide, lath structures in the β matrix (Figure 6b). The intensity of the diffuse streaking in the associated diffraction pattern (Figure 6a) decreased, as did the intensity of the ω reflections when compared to the as-quenched sample. In addition, new areas of increased intensity were observed at the 2/3 <112>β position. An example is ringed in Figure 6a. The lath morphology of the structures shown in Figure 6b combined with both in-situ and ex-situ X-ray diffraction data would suggest that these new reflections correspond to α phase particles. </p><p>A similar observation was made by Sukedai in Ti-20Mo [21]. HRTEM studies showed the lattice around a needle like ω particle contained regions of both α and a transitional state. Unlike Sukedai, the ω reflections reported in the current study do not overlap with the α reflections and therefore are able to confirm the in-situ diffraction data which indicated that ω is present when α first begins to form. </p><p>The lath-shaped precipitates continue to develop when aged for 10 minutes, Figure 7a, which shows precipitates approximately 300 nm in length by 20 nm wide. The continued growth of the precipitates at the aging temperature employed in this study correlates well with the ex-situ X-ray diffraction data presented in Figure 4 and is consistent with the hypothesis that these precipitates are the α phase. Furthermore, electron diffraction from the [110]β zone axis (Figure 7b) shows reflections in addition to those of the matrix and diffuse streaking, which correspond to one Burgers variant of the α phase, illustrated by the corresponding key diagram (Figure 7c). The laths shown in Figure 7a are orientated with their major axis aligned to the [112]β, which is the normal growth direction for the α phase [21]. Why one particular variant would form preferentially during the early stages of development is not clear at this stage and forms the subject of further investigation.</p><p>Following 15 minutes of aging the α laths were observed to increase in size (Figure 8a) to ~ 500 by 25 nm. The bright field image appears to show two orientations of α laths, however a β grain boundary can be identified running across the image, with each orientation contained within separate grains. The corresponding diffraction pattern (Figure 8b) still shows diffuse streaking, however the intensity maxima remain in positions corresponding to a single Burgers variant of α. The continued presence of diffuse streaking suggests that some ω-like structures persist for a significant time period and as observed by Sukedai transitional states between ω and α may well be present. </p><p>The α phase laths continued to develop during aging to 30 minutes, to ~1.5 µm in length by 200 nm wide (Figure 9a). The α laths were evenly distributed and two distinct orientations can be seen within the microstructure. This observation is supported by diffraction from the [110]β zone axis (Figure 9b), which shows reflections consistent with both the expected α variants obeying the Burgers relationship as indicated in the key diagram (Figure 9c). The extra reflections that are observed in Figure 9b, when compared to the key diagram, are due to double diffraction. An α lattice parameter of 2.88 Ǻ can be calculated from Figure 9b, which is in good agreement of that from the in-situ data, which when approximated by a Voigt function produces an α lattice parameter of 2.83 Ǻ.</p><p>The development of the α precipitates during aging results in an associated change in the mechanical properties. Figure 10 shows representative mechanical data, in the form of Vickers hardness, at each aging step. As α develops the hardness increases, suggesting that the final properties of the material are dependant on the α structure. It would also indicate that with control of the aging regime further optimisation is possible through the control of the α development from the pre-existing ω phase.</p><p>Conclusions</p><p>The use of in-situ X-ray synchrotron diffraction combined with ex-situ conventional laboratory X-ray diffraction and transmission electron microscopy have enabled new insights to be gained into the complex phase transformations involved in β phase decomposition of the near β alloy Ti-5-5-5-3.</p><p>In-situ diffraction performed during rapid cooling from above the β transus found evidence to support the concept that embryonic ω structures exist at high temperatures as linear defects. As the temperature decreases the defects correlate and diffract before becoming more discrete once the temperature falls below ~500°C. The presence of ω structures is confirmed by electron diffraction; however the reflections are shifted away from the commensurate positions.</p><p>The relevant timescales involved in the initial stages of α formation during aging of rapidly cooled material containing ω has also been evaluated. Nucleation of α occurs following ~90 seconds spent at the aging temperature and appears to form as a single variant. This single variant grows in a lath morphology, reaching a size of 500 by 25 nm following 15 minutes of aging. During this time period diffuse streaking is visible in all electron diffraction patterns, suggesting that ω persists for significant time periods.</p><p>Further aging results in continued growth of the α phase with laths reaching 1.5 by 0.2 µm in size following 30 minutes at 570°C. Between 15 and 30 minutes aging the second expected α variant for the given (110)β plane nucleates and can be clearly seen in the microstructure. Diffuse streaking is also no longer visible in the electron diffractions pattern, leaving only equilibrium phases present.</p><p>The aging of ω containing material, under normal industrial α aging conditions, has been shown to produce small evenly distributed α laths relatively quickly and therefore this technique could be utilised in further attempts to engineer near β alloy microstructures. The ω structure appears to have no effect on the mechanical properties, which show improvement as the α laths develop. </p><p>Acknowledgements</p><p>The authors would like to acknowledge the provision of funding by EPSRC (DTA studentship for NGJ, GR/T26344/01) and the provision of beamtime at ESRF. The assistance of Seema Raghunathan, Russell Talling and John Daniels in performing the diffraction experiments and analysis is gratefully acknowledged. The assistance of Christabel Evans in helping with TEM specimen preparation is acknowledged. The material used was generously supplied by T Sabin at Messier Dowty. </p><p>References</p><p>[1] Hasegawa A, Ishigai S, Matsushita T. Near Net Shape Forging of Titanium Alloys. In: Lacombe P, Tricot R, Beranger G, editors. Sixth World Conference on Titanium. France: Les Editions de Physique, Les Ulis, 1988. p.1263 [2] Jackson M, Dashwood R, Christodoulou L, Flower HM. Metall. Mater. Trans. A 2005;36:1317. [3] Jones NG, Dashwood RJ, Dye D, Jackson M. Mater. Sci. Eng., A 2008. [4] Boyer RR, Briggs RD. J. Mater. Eng. Perform. 2005;14:681. [5] Fanning J. JOM 2004;56:112. [6] Duerig TW, Williams JC. Overview: Microstructure and Properties of Beta Titanium Alloys. In: Boyer RR, Rosenberg HW, editors. Beta Titanium Alloys in the 1980's. Altanta, Gerogia: The Metallurgical Society of AIME, 1983. [7] Raghunathan SL, Stapleton AM, Dashwood RJ, Jackson M, Dye D. Acta Mater. 2007;55:6861. [8] Ohmori Y, Toshitaka O, Kiyomichi N, Kobayashi S. Mater. Sci. Eng., A 2001;312:182 [9] Clement N, Lenain A, Jacques PJ. JOM 2007;59:50 [10] Cotton JD, Boyer RR, Briggs RD, Baggerly RG, Meyer CA, Carter MD, Wood W, Tewksbury G, Li V, Yao X. Phase Transformations in Ti-5Al-5Mo-5V-3Cr-0.5Cr. In: Ninomi M, Akiyama S, Ikeda M, Hagiwara H, Maruyama K, editors. Ti - 2007: Science and Technology. Kyoto, 2007. [11] Sikka SK, Vohra YK, Chidambaram R. Prog. Mater Sci. 1982;27:245 [12] Banerjee S, Tewari R, Dey GK. Int. J. Mat. Res. 2006;97:963 [13] Sanchez JM, De Fontaine D. Acta Mater. 1978;26:1083. [14] Williams JC, De Fontaine D, Paton NE. Met. Trans 1973;4:2701 [15] Schryvers D, Tanner LE. Mater. Sci. Forum 1990;56 - 58:329. [16] Burgers WG. Physica 1934;1:561. [17] De Fontaine D, Paton NE, Williams JC. Acta Mater. 1971;19. [18] Ivasishin OM, Markovsky PE, Semiatin SL, Ward CH. Mater. Sci. Eng., A 2005;405:296. [19] Gysler A, Luetjering G, Gerold V. Acta Metall. 1974;22:901 [20] Sinkler W, Luzzi DE. Acta Mater. 1994;42:1249 [21] Sukedai E. Alpha-Phase Structure Observed in a Needle Shape Omega-Phase Precipitate formed by a Two-Step Aging Method in a Beta-Type Ti-Mo Alloy. In: Ninomi M, Akiyama S, Ikeda M, Hagiwara H, Maruyama K, editors. Titanium '07: Science and Technology, vol. 1. Kyoto, Japan, 2007. p.455 V Fe Al Mo Cr C O N H Ti-5-5-5-3 5.25 0.35 4.91 5.22 2.98 0.008 0.135 0.006 0.001 Table 1: Chemical composition (wt. %) of the Ti-5-5-5-3 alloy investigated. Figure 1: Schematic time-temperature plot of the quench and aging performed during the synchrotron X-ray diffraction experiment and corresponding diffraction data obtained at selected points in the thermal treatment sequence. Figure 2: In situ X-ray diffraction data obtained during rapid cooling of Ti-5-5-5-3 from 950°C to ~50°C around the β(110) peak. Figure 3: In situ X-ray diffraction data around the β (110) peak, obtained during the aging of Ti-5-5-5-3 at 570°C following rapid cooling from 950°C. Figure 4: Ex situ laboratory X-ray diffraction results for Ti-5-5-5-3 quenched from 900°C and aged at 570°C for 1, 3, 5, 10, 15 and 30 minutes. Figure 5: a) Electron diffraction pattern from [110]β zone axis (sample quenched from 900°C, camera length D = 800mm), b) electron diffraction pattern from the [110]β zone (D = 2000 mm) and c) schematic representation of the diffraction pattern showing movement of ω reflections towards the octahedral site. Figure 6: a) Electron diffraction pattern from [110]β zone axis (sample quenched from 900°C and aged for 5 minutes at 570°C, D = 800 mm) and b) corresponding bright field image showing several α lath structures. Figure 7: a) Bright field image of α laths observed in a sample quenched from 900°C and aged for 10 minutes at 570°C, b) corresponding electron diffraction pattern from the [110]β zone axis, D = 800 mm and c) key diagram for the [110]β zone axis diffraction pattern with reflections from a single α variant overlaid. Figure 8: a) Bright field image of α laths in a sample quenched from 900°C and aged for 15 minutes at 570°C (note a β grain boundary traversing the image from left to right), b) corresponding electron diffraction pattern from the [110]β zone axis, D = 800 mm. Figure 9: a) Bright field image showing two α lath variants in a sample quenched from 900°C and aged for 30 minutes at 570°C, b) corresponding electron diffraction pattern from the [110]β zone axis, D = 800 mm and c) key diagram of [110]β zone axis with reflections corresponding to two α variants overlaid. Figure 10: Evolution of sample hardness with aging time (the line is a guide to the eye).</p>
Details
-
File Typepdf
-
Upload Time-
-
Content LanguagesEnglish
-
Upload UserAnonymous/Not logged-in
-
File Pages21 Page
-
File Size-