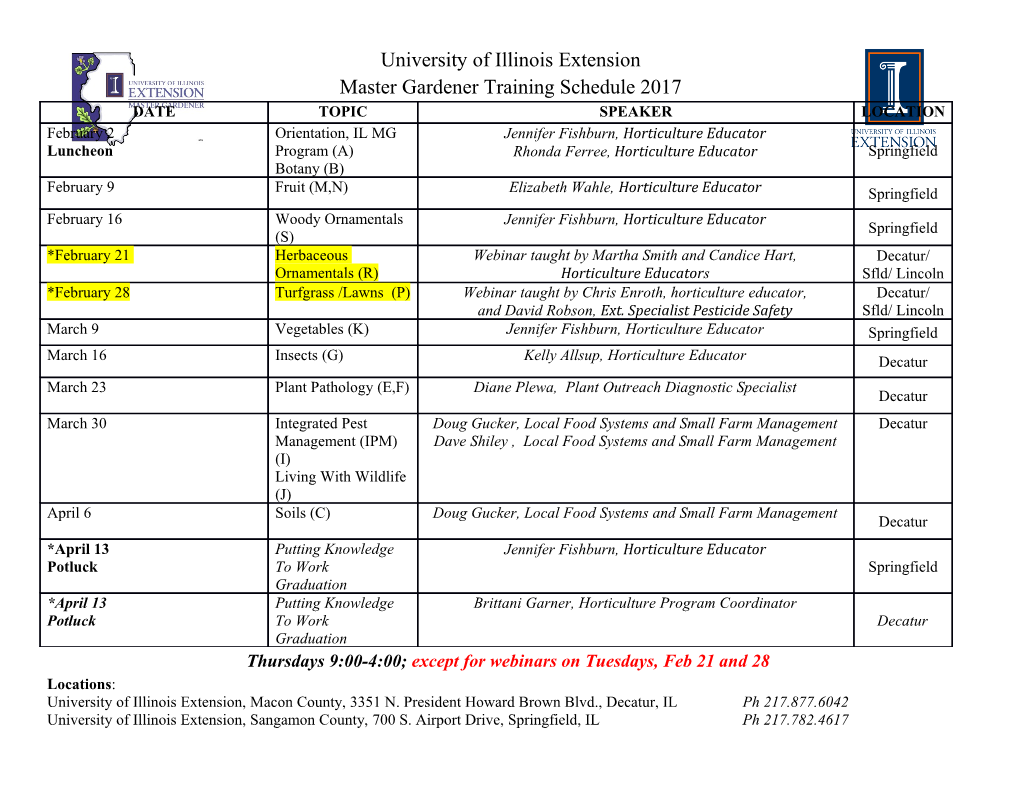
<p> SUBARACHNOID HEMORRHAGE TRAUMATIC WILLIAM A. COX, M.D. FORENSIC PATHOLOGIST/NEUROPATHOLOGIST</p><p>November 3, 2009</p><p>General Information Bleeding into the subarachnoid space is most commonly the result of trauma. It can present either as a thin-layered diffuse hemorrhage over the cerebral or cerebellar hemispheres or assume a more patchy distribution. The origin of these hemorrhages is from small extracerebral or cerebellar vessels most likely veins, which have been subjected to shearing stress. Subarachnoid hemorrhage over the dorsal surface of the cerebellar hemispheres is especially frequent. Hematomas within the subarachnoid space are space occupying, usually approximately 50 cc of blood, and due generally to tears in small to large extracerebral arteries. As stated above, the subarachnoid hemorrhage may be confined to focal areas, which have been exposed to stresses during impact such as the frontal poles in an individual, who falls and strikes the back of his head giving rise to contracoup stresses. Focal subarachnoid hemorrhage can be seen on the surface of the hemispheres directly below the point of impact or fracture. Also, how acute subarachnoid hemorrhage represents itself may be determined when the person dies. If the person dies immediately, multiple focal areas of hemorrhage can be seen suggesting a multiplicity of bleeding spots. However, if the person should survive for a period of time, the multifocal areas of hemorrhage may coalesce forming a thin rim of acute subarachnoid hemorrhage, which has spread over the surfaces of the hemispheres, with a tendency to accumulate along the entrances to the sulci. Subarachnoid hemorrhages associated with cortical contusions tend to be more extensive and may form a hematoma, which, if there is an existing tear in the arachnoid may give rise to hemorrhage into the subdural space. Traumatic subarachnoid hemorrhage typically occurs in association with other traumatic injuries to the brain such as epidural and subdural hematomas, contusions, intraparenchymal hemorrhage and diffuse axonal injury. Although, subarachnoid hemorrhages are generally non-space occupying, there is an association between the presence of subarachnoid hemorrhage and a poor outcome. This poor outcome is related more to the associated traumatic injuries than the acute subarachnoid hemorrhage itself or its consequences in the form of vasospasm with its attendant ischemia. Also, as is the case with acute subdural hemorrhages, you cannot use the location of an acute subarachnoid hemorrhage to determine the site of impact. The actual site of impact is best determined through utilization of external injuries, scalp hematomas, skull fractures and contusions. Morphologic (Gross and Microscopic) Appearance Initially these hemorrhages present as a thin rim of hemorrhage, without evidence of coagulation, due primarily to the dilution by cerebral spinal fluid (CSF). Small hematomas associated with cerebral contusions may coagulate due to the large quantity of red blood cells (RBC) vs. the quantity of CSF. The initial red color of acute subarachnoid hemorrhage becomes darker over the ensuing days eventually turning a purplish-black color. After approximately one week it assumes a brown color. This color change is very dependent on the thickness of the hemorrhage because the thicker the bleeding site the apt it is to retain its black color for a longer period of time. Overtime the brown color changes into a yellow color, which may persist for months depending on the original amount of blood present as well as the quantity of CSF that the hemorrhage is exposed to. Typically the subarachnoid hemorrhage should completely disappear in 6 to 12 months. However, in those subarachnoid hemorrhages, which occur in association with cortical contusions the leptomeninges may remain stained for several years. You may see thickening of the leptomeninges as well as scar formation within the subarachnoid space with cyst formation, however, cyst formation occurs rarely. At the microscopic level the initial reaction to the RBC within the subarachnoid space is the appearance of leukocytes from the congested veins after a few hours of the event. If the blood is well mixed with CSF, lysis of RBC may occur within the first two days, however intact RBC can sometimes be seen after a week. </p><p>2 The first reaction of the mesothelial cells of the subarachnoid trabeculae is swelling of their nuclei, which can be seen within 12 hours of the event becoming very evident within 24 to 48 hours. The first phagocytes usually do not appear before the second day, with some containing hemosiderin. It is the cells forming the pia that give rise to the macrophages rather than the cells forming the arachnoid. Depending on the quantity of the hemorrhage, the formation of the phagocytes typically reaches their maximum after 2 to 4 weeks. After about 6 to 8 weeks, the subarachnoid space becomes gradually cleared and the subarachnoid tissue undergoes regressive changes so that eventually no scar develops except for some thickening of the connective tissue layer near the arachnoid proper. Single phagocytes containing hemosiderin can be found in the delicate connective tissue framework for years. Even if all of the phagocytes have disappeared, hemosiderin can still be found in the superficial glia of the first cortical layer for years, constituting the only evidence of a remote subarachnoid hemorrhage. Also, the subarachnoid hemorrhage may extend into spaces around the pacchinonian granulations where they are attached to the dural sinuses; thus, when the RBCs have been lysed you may see residual hemosiderin containing phagocytes. The bleeding around the pacchianian granulations can also give rise to another problem and that is the formation of fibrous tissue with its consequent impediment of the absorption of CSF and thus a communicative hydrocephalus. What is important to keep in mind is that the appearance of subarachnoid hemorrhage can occur as a postmortem artifact. This occurs when the brain is removed, the resulting tearing of the cerebral veins and arachnoid can give rise to focal areas of subarachnoid hemorrhage. This happens usually along the dorsal margin of the cerebral hemispheres, over the temporal poles, the posterior aspect of the inferior temporal gyrus, and the dorsum of the cerebellum. There is another artifact that you need to be aware of and that is bleeding into the subarachnoid space from a focus of acute hemorrhagic infarction involving the cortex. If you examine the cortex closely you will see it is soft, swollen and hemorrhagic and that the dark color of the overlying leptomeninges is due to the underlying hemorrhagic cortex rather than blood actually accumulating in the subarachnoid spaces.</p><p>3 Subarachnoid Hematomas Subarachnoid hematoma is a localized mass of blood and as such is space occupying with the potential to exert mass effect if it attains a mass of at least 50 cc. In contradistinction to this, subarachnoid hemorrhage consists of a thin rim of diffuse hemorrhage or patches of acute hemorrhage, and as such it is not space occupying, thus will not exert mass effect. Subarachnoid hematomas typically occur as a focal lesion usually in association with acute contusions of the cortex. They can occur at the base of the brain or over the convexity of the cerebral hemispheres. It is felt these lesions arise due to tears in a large vein or in an artery. On a rare occasion subarachnoid hematoma may arise in a pre- existing arachnoidal cyst. Acute Subarachnoid Hemorrhage at the Base of the Brain Some believe extensive bleeding into the cisterns at the base of the brain is a separate distinct type of subarachnoid hemorrhage. In their gross appearance they are very similar in appearance to the acute subarachnoid hemorrhage occurring at the base of the brain due to rupture of an aneurysm of the circle of Willis. There is a common threading that runs through these cases and that is they involve a blow to the face, forehead or side of the neck. Some have reported that in many of these cases the arteries at the base of the brain were thin-walled and some were hypoplastic. Typically these cases show no evidence of fracture and or acute contusions. Fraenckel has reported cases of this nature as early as in 1927 and Wolf in 1927. In 1963 Simonsen reported in the Journal of Forensic Science that most of his cases as well as the cases of others were intoxicated at the time of injury. It was also his opinion that the arteries involved were not thin-walled or hypoplastic, but normal. Ford in 1956 published an article in the Journal of Forensic Science that in his experience the external force applied was so innocuous that he believed that some of the acute traumatic induced basilar hemorrhages were the result of a sudden increase in systolic blood pressure due to the physical exertion or emotional stress or both. Fretag, however, published an article in the Archives of Pathology in 1963 in which she believed that trauma was more important for it gave rise to brain oscillations which caused a tear in an artery at the base of the brain. One of her most interesting cases was that of a 4 year-old boy who was struck on the right side of his neck beneath </p><p>4 the right ear by a 2-oz lead weight on a fishing line. Although, the carotid artery beneath the impact area showed no pathologic change, the boy developed a basal acute subarachnoid hemorrhage. It was Fretag’s belief the force of the impact of the 2-oz lead weight was transmitted through the right carotid artery into the vasculature at the base of the brain, giving rise to a rupture of one of the vessels. Typically what these cases show is damage to a vessel in the vertebrobasilar vascular system or one of their branches. However, the actual vessel that is torn is often very difficult to identify. Having said that, in 1955 Krauland reported a series of cases in which complete or partial tears were seen in the carotid, basilar, and other arteries at the base of the brain. Krauland also made an interesting observation of the development of aneurysms in vessels with incomplete tears. Richard Lindenberg reported on five cases in which one involved the intracranial carotid artery, one the anterior cerebral artery, one the posterior communicating artery, one the posterior cerebral artery and one vertebral artery. On a rare occasion the hematoma may be produced by a tear in a large vein such as one of the internal cerebral veins where they enter into the great vein of Galen or actually arise from the great vein of Galen where it is still within the subarachnoid space of the transverse cistern. Alcohol and Traumatic Acute Subarachnoid Hemorrhage Simonsen’s observation that in most of his cases, as well as those of others, the patient was intoxicated at the time of the event is not an uncommon scenario in those cases that come to the medical examiners or coroner’s office. Whether alcohol directly affects vascular wall integrity is not known. However, it has been shown that red wine strongly inhibits the synthesis of endothelin-1, which is a vasoactive peptide that is important in the development of coronary atherosclerosis and hypertension. Endothelins are 21 amino-acid peptides that are produced by the endothelium. Release of endothelin-1 by the endothelium gives rise to vasoconstriction as well as influencing local cellular growth. Receptors for endothelin are also found in cells of the brain, choroids plexus and peripheral nerves. Direct application of endothelin-1 to the surface of the brain causes pronounced metabolic stimulation and seizures along with decrease blood flow to those regions of the brain with both of these effects being mediated by calcium channels. Thus,</p><p>5 it would appear the presence of alcohol in mild to moderate quantities would appear to enhance vascular dilatation and thus enhance the possibility of blood loss through a complete or partial tear in the vessel wall. Moderate alcohol consumption also decreases coagulation factors, thus enhancing bleeding, through decreasing clot formation. Moderate alcohol intake reduces platelet aggregation, fibrinogen levels, plasma viscosity, von Willebrand factor and Factor VII. Von Willebrand factor (vWF) is a glycoprotein whose primary function is to bind to other proteins, especially Factor VIII, which degrades rapidly when no longer bound to vWF. VWF is also important in the development of platelet adhesion at wound sites. What is of particular interest is vWF appears to be most efficient in defects, which develop in narrow blood vessels with high flow (high shear stress). It appears the vWF has the ability to uncoil under these circumstances, thus trapping or if you will decelerate the passing platelets, thus enhancing platelet adhesion to the vascular defect. It would appear that alcohols enhancement of vascular dilatation and its inhibition of coagulation promote hemorrhage from vessels that have either a partial or complete tear of their wall. Morbidity and Mortality from SAH According to Schievink et al, 2004, approximately 10/100,000 people suffer from aneurysmal SAH. Schievink in 1997 reported that SAH has a mortality of 12% before receiving medical attention, with a further 40% dying within a month of admission to the hospital. Of the survivors 30% will show morbidity as reported by Sekhar et al, 1988; Grosset et al, 1992; Sobey and Faraci, 1998; and Kaptain et al, 2000. As indicated in the previous article concerning non-traumatic acute SAH, it has been the general belief that the most important cause for morbidity and mortality following SAH is vasospasm of the cerebral arteries with its consequent cerebral ischemia and infarction. The underlying mechanism for the vasospasm has hitherto-to-for been attributed to the increase in K ion within the CSF due to lysis of RBC, inflammatory mediators, the released vasoconstrictive agents and the inhibitors of vasodilating agents. However, recent research has indicated that this mechanism may be in actuality to simplistic, for it does not take into consideration apoptosis, which a number of studies show may play a significant role in the pathogenesis of secondary brain injury after SAH.</p><p>6 When you examine the underlying causation of early brain injury (EBI), which refers to the immediate brain injury, that is brain injury occurring within the first 72 hours after the event, it occurs before the development of vasospasm. Hence, vasospasm cannot be considered the underlying cause of EBI. Having said this, this does not mean that the etiology of vasospasm is not connected to EBI. EBI is a reflection of immediate injury whereas vasospasm may be a sequela to EBI. How EBI occurs is directly related to the SAH and the consequent initiated pathophysiologic mechanism that arises. For example, at the initiation of the SAH following a rupture of an aneurysm there is an immediate rise in intracranial pressure (ICP) with a concomitant decrease in cerebral blood flow (CBF), the severity of which is determined by volume of the bleed. What the exact mechanism is giving rise to the sudden increase in ICP is not known. Grote and Hassler reported in 1988 that the sudden increase in ICP might be due to an interplay involving an increase in volume in the subarachnoid space (Monroe-Kellie Hypothesis), vasoparalysis and CSF drainage obstruction. What has been suggested is that the decrease in CBF due to the increase in ICP may be a protective mechanism in an attempt to control blood loss from the aneurysm or torn vessel due to trauma. However this protective mechanism is very short lived for it results in a decrease in cerebral perfusion, which gives rise ultimately to hypertension as well as perhaps vasodilatation, which gives rise to an increase in cerebral blood flow. The most important consequence of the increase in ICP is global ischemic injury. This ischemic injury as a result of SAH can be seen in patients postmortem (Bederson et al, 1995) and is seen as a leading cause of morbidity in patients with SAH (Frykholm et al, 2004). Although, the degree of SAH differs among patients, they all experience to varying degrees these changes in cerebral pathophysiology. This fundamental point was clearly demonstrated by the World Federation of Neurological Surgeons when they pointed out that even in patients with a grade one SAH, who do not exhibit clinical or radiological vasospasm, who show no obvious complications due to surgery or in the postoperative course, still show long-term psychological difficulties (Hutter et al, 1999; Dreiter et al, 2002; Claassen et al, 2004). Dreite et al in 2002 reported that among survivors of SAH; up to 50% show some degree of cognitive dysfunction in the long </p><p>7 term. It is clear this ischemic is one of the leading causes of morbidity in patients with SAH. Many believe that the changes in behavior, memory, etc. are the result of EBI and are long-term complications that cannot be explained by vasospasm alone. It has been demonstrated in both animal models and in postmortem studies (Park et al, 2004; Ostrowski et al; 2005 and Zhou et al, 2005) that global ischemic injury due to increase in ICP with its concomitant decrease in CBF gives rise to the genesis of apoptosis throughout the brain following SAH. Kerr et al originally described Apoptosis in 1972, and is the term utilized to describe programmed cell death. It is involved in a number of brain pathologies such as stroke, Alzheimer’s disease, and SAH. Apoptosis is known to occur in the hippocampus, blood brain barrier (BBB), and vasculature with varying degrees of necrosis immediately following global ischemia of SAH (Zubkov et al, 2001; Park et al, 2004; Ostrowski et al, 2005 and Zhou et at, 2005). Zubkov et al reported in 2000 that the development of vasospasm and smooth muscle proliferation in the spastic arteries was due to apoptosis. Apoptosis is also believed to play a role in aneurysmal formation and rupture. What is important to understand is if the ischemia is global the resulting apoptosis can be more severe than the actual ictus, whether that be a rupture of an aneurysm or tearing of a vessel. There are a number of apoptotic pathways that are believed to play a role in SAH: the death receptor pathway, caspase-dependent and –independent pathways, and the mitochondrial pathway. The intracellular mechanisms responsible for apoptysis are similar in all animals; these mechanisms depend on a family of proteases that have cysteine at their active site and cleave their target proteins at specific aspartic acids, hence they are called caspases. Caspases are synthesized in the cell as inactive precursors, or procaspases, which are usually activated by cleavage at aspartic acids by other caspases. Once activated, caspases cleave, and thereby activate, other procaspases, resulting in an amplifying proteolytic cascade. Some of the activated caspases then cleave other key proteins in the cell. Some cleave the nuclear lamins, causing irreversible breakdown of the nuclear lamins; another cleaves a protein that normally holds a DNA-degrading enzyme (αDNAse) in inactive form, freeing the DNAse to cut up the DNA in the cell nucleus.</p><p>8 Nuclear lamins are key regulators of nuclear structure and activities. The nuclear lamina is a proteinaceous structure underneath the inner nuclear membrane (INM) where it associates with the peripheral chromatin. It contains lamins and lamin associated proteins, including many integral proteins of the INM, chromatin modifying proteins, transcriptional repressors and structural proteins. A fraction of the lamins is also present in the nucleoplasm, where it forms stable complexes and is associated with specific nucleoplasmic proteins. The lamins and their associated proteins are required for most nuclear activities, mitosis and for linking the nucleoplasm to all other major cytoskeletal networks in the cytoplasm. Activation of intracellular cell death pathways is usually triggered in a complete, all-or- none fashion. The protease cascade is not only destructive and self-amplifying but also irreversible, so that once a cell reaches a critical point along the path of destruction, it cannot turn back. It is clear from the above that all nucleated animal cells contain the seeds of their own destruction; hence caspase activity is tightly regulated inside the cell to make certain the death program is held in check until it is needed. The question that needs to be asked is how are the procaspases activated to initiate the caspase cascade? It appears, adaptor proteins, which bring together multiple copies of specific procaspases called initiator procaspases, forming a complex or aggregate, bring about the activation. This results in some cases in the initiator procaspases cleaving one another, which triggers their mutual activation. In other cases, the aggregation is thought to cause a conformational change that activates the procaspase. Within seconds, the activated caspase at the beginning of the cascade cleaves downstream procaspases to amplify the death signal and spread it throughout the cell. The activation of the procaspase can be triggered from outside the cell through activation of death receptors on its surface. As an example, killer T lymphocytes, on recognizing a target cell, can induce apoptosis within that cell by producing a protein called a Fas ligand, which then binds to a death receptor protein called Fas located on the surface of the target cell. The clustered Fas proteins then recruit intracellular adaptor proteins, which were discussed above, which bind and aggregate procaspase-8molecules, which </p><p>9 cleave and activate one another. The activated procaspase-8molecules then activate downstream procaspases to induce apoptosis. There are also instances in which a stressed or damaged cell will kill itself through extracellular activation by producing both the Fas ligand and the Fas protein, thereby triggering an intracellular caspase cascade without ever acquiring the assistance of a killer T lymphocyte to initiate the process. Stressed or damaged cells can also kill themselves through intracellular activation by inducing their mitochondria to release the electron carrier protein cytochrome с into the cytoplasm, where it binds to and thus activates an adaptor protein Apaf-1 (apoptotic peptidase activating factor 1). Apaf-1 binds to cytochrome c and dATP forming an oligomeric apoptosome. The apoptosome binds and cleaves procaspase-9 molecules, releasing its activated form, caspase 9, which leads to the cleavage of these molecules and the triggering of a caspase cascade. The next question that needs to be asked is there a mechanism in place that regulates the activation of procaspases and thus the initiation of the dell death program? In actuality there is and it exist in two forms: Bcl-2 family of intracellular proteins and the IAP (inhibitor of apoptosis) family of proteins. Whereas the activation of the death receptors on the cell surface via Fas ligand and Fas proteins induces the extracellular apoptotic pathway, the intrinsic apoptotic pathway is dominated by the Bcl-2 family of proteins, which governs the release of cytochrome c from the mitochondria. Some members of the Bcl-2 family of proteins are proapoptotic (they initiate apoptosis), whereas others are antiapoptotic (they inhibit apoptosis). The proapoptotic members are Bax, Bad, Bid, Bik, Bak, Bcl-Xs, and Bim. Bcl-2, Bcl-X2, and Mcl-1 are antiapoptotic. Bcl-2, Bcl-X2, and Mcl-1 inhibit apoptosis in part by blocking the release of cytochrome c from the mitochondria doing this by binding to Apaf-1. On the other hand Bad binds to and thus inactivates the death-inhibiting members of the family, whereas Bax and Bak, stimulate the release of cytochrome c from the mitochondria. Bax and Bak are themselves activated by other apoptosis-promoting members of the Bcl family such as Bid. What is of interest is if the genes encoding for Bax and Bak are both inactivated, the cells are remarkably resistant to most apoptosis-inducing stimuli.</p><p>10 The IAP family of proteins inhibits apoptosis in two ways: they bind to procaspases to prevent their activation, and they bind to caspases to inhibit their activity. What is of interest and underscores the importance of the IAP family of proteins in inhibiting apoptosis is that when mitochondria release cytochrome c to activate Apaf-1, they also release a protein that blocks IAPs. In the final analysis it is the overall ratio of pro- to antiapoptotic signals that finally determines whether or not a cell dies. It does appear that what particular apoptotic cascade takes place is somewhat dependent on the initiating event. For example, ischemia appears to favor initiation of the caspase- dependent cascade, whereas neurotoxins tend to initiate the caspase-independent cascade. The most important cascade following SAH is the initiation of the death receptor/p53 pathway, which was described by Zhou et al in 2005. What is important to understand is once one of these cascades is initiated, at some point all of the cascades become involved due to the fact the proteins involved in each are intimately interwoven. In regard to SAH, the death receptors Fas, TNFR, and DR3-5 located in the cell membrane give rise to the translation of the death signal across the cell membrane through the activation of the tumor necrosis factor receptor (TNFR) family. It is the TNFR family that is the primary focus in SAH. The TNFR family includes tumor necrosis factor-α (TNFRα), TNF-related apoptosis-inducing ligand, and the Fas ligand. It has been shown by Zhou et al that TNR-α is up regulated by SAH. It is these factors/ligands, which are also referred to as cytokines, which through some as yet unknown mechanism provide the connection between the ictus of SAH and the evolution of the apoptotic cascades. It appears that the stress induced on the cell by acute SAH gives rise to a signal that travels across the cell membrane into the cytoplasm. This transduction of the stress signal is dependent primarily on TNF-α and the Fas ligand. TNR-α is a cytokine involved in inflammation and is a member of a group of cytokines that stimulate the acute phase reaction. The primary role of TNF-α is in the regulation of immune cells, however, it is also able to induce apoptotic cell death, induce inflammation, and to inhibit tumorigenesis and viral replication. TNR-α activates two types of receptors, TNF-R1 and TNF-R2. TNF-R1 is found in most tissues, whereas </p><p>11 TNF-R2 is found in cells of the immune system. In regard to the induction of apoptosis (the death signal) it is the activation of TNF-R1 that is important. TNF-R1 binds to TRADD (tumor necrosis factor receptor type 1-associated death domain protein), which in turn binds to FADD, an adaptor protein, that results in the cleavage of procaspase-8 molecules giving rise to activated caspase 8 molecules. Caspase 8 in turn activates Bid, which translocates to the mitochondria inducing cytochrome c release. Once in the cytoplasm, cytochrome c binds to and activates the adaptor protein, Apaf-1. Apaf-1 in turn binds and aggregates procaspase-9 molecules, which leads to the cleavage of these molecules and the triggering of a caspase cascade. The Fas ligand (FasL or CD95L) is a type of transmembrane protein. The binding of Fas ligand with the death receptor protein Fas gives rise to the recruitment of intracellular adaptor proteins, such as FADD, that bind and aggregate procaspase-8molecules, cleaving them, giving rise to activated caspase 8. The activated caspase-8 molecules than activate downstream procaspases to induce apoptosis. One of the key players in the cellular response to stress, such as that induced by SAH is p53. p53 is a protein, referred to as a transcription factor. Transcription factors are proteins, which bind to specific DNA sequences, thereby controlling the transfer (transcription) of genetic information from DNA to mRNA (messenger RNA) via RNA polymerase, which makes the copy of the gene on the DNA molecule transferring it to mRNA. The mRNA then carries the coded information to the site of protein synthesis. A transcription factor can perform this function alone or with other proteins in a complex, by promoting (as an activator), or blocking (as a repressor) the recruitment of RNA polymerase, the enzyme that as indicated above, actually performs the transfer (transcription) of genetic information from DNA to mRNA. There are other proteins, which can aid the transcription factors in their function, such as coactivators (a protein which works with transcription factors to increase the rate of gene transcription), chromatin remodelers, histone acetylases, etc. p53 gets its name from its name from its apparent molecular mass, which was believed to be 53 kilodactons. However, based on calculations from its 393 amino acid residues the molecular mass of p53 is really 43.7 kDa.</p><p>12 p53 is produced in the nucleus. It is transported from the nucleus to the cytosol of the cell, where it remains in very low concentration, because it is unstable and is quickly degraded. The p53 inhibitor Hdm2 is largely responsible for keeping p53 in this state. Hdm2 does this through inhibition of p53 transcriptional activity and more importantly by promoting its degradation within the cytosol through proteasomes. Proteasomes are large protein complexes found within the nucleus and the cytoplasm whose main function is to degrade unneeded or damaged proteins by proteolysis. The enzymes, which carry out these reactions, are called proteases. Proteasomes are part of a major mechanism by which cells regulate the concentration of particular proteins and degrade misfolded proteins. P53 has many functions which include activation of DNA repair proteins when DNA has sustained damage; induce growth arrest by holding the cell cycle at G1/S regulation point on DNA damage recognition (if it holds the cell here for long enough, the DNA repair proteins will have time to fix the damage and the cell will be allowed to continue the cell cycle); it can initiate apoptosis, if the DNA damage proves to be irreparable and if the cell is subjected to stress The normal existence of p53 within the cell is drastically changed when the cell is exposed to stress, such as that induced by SAH. Such stress leads to the stabilization of p53 through phosphorylation and thus its accumulation within the nucleus. It is believed that the accumulation of p53 in the nucleus leads to translocation of p53 into the cytoplasm (Sansome et al, 2001; Chipuk et al, 2004; Erster et al, 2004). Once in the cytoplasm it acts directly or indirectly on the mitochondria, mechanism unknown, causing the release of cytochrome c. This concept is in part based on the fact that SAH does not cause DNA damage, therefore the induce apoptosis is believed to be due to cytoplasmic p53 acting directly on the mitochondria or indirectly through an intermediate such as Bax. Once in the cytoplasm cytochrome c forms a complex with Apaf-1 and procaspase-9 called apoptosome as described above, in which caspase-9 is activated, which in turn causes the activation of caspase-3, caspase-6, caspase-7 and caspase-8. p53 is thus involved in both the extrinsic and intrinsic apoptotic pathways with both of these converging through Bid.</p><p>13 What is the relationship between apoptosis and mitochondria? During apoptosis cytochrome c is released from the mitochondria into the cytoplasm. Cytochrome c is a transcription protein that is located in the mitochondrial intermembranous space. During apoptosis, this membrane becomes permeable to cytochrome c, possibly through pore formation or membrane destruction. As stated above, once cytochrome c is released into the cytosol it binds to Apaf-1. The resulting complex, apoptosome, recruits and cleaves procaspase-9, which as has been previously discussed, activates the downstream caspase cascade. The most critical step in this sequence is that of the release of cytochrome c, which is mediated by the Bcl-2 family, which in turn is controlled by p53. The importance of p53 in this process was demonstrated by the inhibition of p53, which in turn arrested the translocation of cytochrome c from the mitochondria. Similarly, caspase-8 was shown to decrease in experimental modules of SAH-induced apoptosis after the prevention of p53 stabilization in the cytoplasm, suggesting that the caspase- dependent pathway and mitochondrial release of cytochrome c are important in cellular stress due to SAH. As discussed above, it is clear p53 plays a central role in apoptotic caspase-dependent pathways in SAH. Recently it has been demonstrated that p53 also plays a role in apoptotic caspase-independent pathways. This in part came about through the observation as noted in the above paragraph that although inhibition of caspases in the apoptotic caspase-dependent pathways induced by SAH affords some protection; apoptosis still occurs. Therefore, it is rather clear that a caspase-independent cascade is also involved in the cellular stress induced by SAH. To further support this concept, located within the mitochondrial intramembranous complex is a flavoprotein referred to as apoptosis-inducing factor (AIF) that has been shown to be released from the mitochondria and translocated to the nucleus in response to various death signals (Cregan et al, 2002). p53 has been shown to trigger the release of AIF in the absence of Apaf-1, resulting in a caspase-independent apoptotic cascade (Cregan et al, 2002). What is also of interest, is that like cytochrome c, AIF appears to be under the control of Bcl-2 family and in fact the release of both AIF and cytochrome c is inhibited if Bcl-2 members are blocked, suggesting that the Bcl-2 family may be solely responsible for caspase- dependent and –independent cascades (Cregan et al, 2002). Bcl-2 family is also </p><p>14 responsible for the inhibition of a second mitochondrial-derived activator of caspase/direct IAP (inhibitor of apoptosis protein) binding protein with low pI. This mitochondrial protein is similar to citochrome c, which depresses procaspase-9 through the inhibition of inhibitor of apoptosis protein. This makes the Bcl-2 family a powerful target for therapeutic intervention. Consequences of Apoptosis One of the consequences of the above-discussed apoptotic cascades induced by SAH is disruption of the blood brain barrier (BBB) (Germano et al, 2000; Kusaka et al, 2004; Park et al, 2004). It appears based in part on experimental evidence that the disruption of the BBB due to SAH is a biphasic phenomenon with the changes occurring between 1 hour and 6 days. The early effects on the BBB are due to pathophysiologic disturbances initiated by SAH, whereas the apoptotic cascades mediate the later effects. The early-enhanced BBB permeability experimentally has been shown to be related to activation of several intracellular signaling pathways, including tyrosine kinase and its substrate mitogen-activated protein kinase (MAPK) pathways (Fujikawa et al, 1999; Tibbs et al, 2000; Vollrath et al, 1998). Tyrosine and MAPK regulate tissue stress response, which leads to cellular differentiation, cell proliferation, cell death, or cell survival. Tyrosine kinase is an enzyme that transfers a phosphate group from ATP to a tyrosine residue in a protein, hence its name. Kinases in general are enzymes that transfer phosphate groups from high-energy donor molecules, such as ATP, to specific substrates. This process is called phosphorylation. In contradistinction to this an enzyme that removes a phosphate group is known as a phosphatase. One of the largest groups of kinases or phosphorylases is protein kinases of which tyrosine kinase is a member. A protein kinase is thus a kinase enzyme that modifies other proteins by adding phosphate groups to them (phosphorylation), which results in a functional change in the protein. Tyrosine protein kinases phosphorylate tyrosine amino acid residues and are involved in signal transduction. They act primarily as growth factor receptors and in downstream signaling from growth factors. Mitogen-activated protein kinases are serine/threonine-specific protein kinases that respond to extracellular stimuli (mitogens, which are chemical substances that </p><p>15 encourages a cell to commence with division) and regulate various cellular activities, such as gene expression, mitosis, differentiation, proliferation, and cell survival/apoptosis. There are six distinct groups of MAPKs. The three major subgroups, which are activated as the result of SAH, are extracellular signal-regulated kinase (ERK), c-jun-n-terminal kinase (JNK), and p38. These MAPKs are activated by stress stimuli, such as cytokines and growth factors including vascular endothelial growth factor (VEGF). VEGF is an endothelial mitogen, which is expressed in neuronal and vascular tissues in the brain (Ogunshola et al, 2002). VEGF binds with its receptor (a receptor tyrosine kinase) and activates MAPK signaling pathways. The levels of VEGF in the CSF as well as its expression in brain tissues are increased in SAH, which is believed to enhance BBB permeability. One of the things that must be kept in mind is that with enhanced vascular permeability, there is activation of the mitochondrial apoptotic cascade, which gives rise to the late effect of disruption of the BBB. This activation of the apoptotic cascade occurs primarily in the cerebral endothelial cells, partially in neurons in the hippocampus, and to a lesser degree in the cerebral cortex. Damage to the endothelial cells in addition to leading to further BBB disruption, may also decrease the production of endothelial-dependent relaxing factors, which has been speculated to make a contribution to cerebral vasospasm. This is further exacerbated through the death of the endothelial cells due to apoptosis and thus denuding the vessels of endothelial cells, which exposes the vessel to a myriad of vasoactive and toxic metabolites, which further contributes to the vasospasm. It is this disruption of the BBB and subsequent edema that have been implicated as one of the major predicators of cognitive dysfunction as long-term consequence of SAH (Kreiter et al, 2002). One of the direct consequences of the disruption of the BBB is brain edema, which is one of the major components of EBI. Claassen et al, 2002 demonstrated that global cerebral edema occurs in 8% of patients with SAH as an early manifestation with an additional 12% developing appreciable edema over the next 6 days. In patients with SAH, classic vasogenic edema occurs as a direct result of disruption of the BBB (Doczi, 1985). However, it has also been recently demonstrated that cytotoxic edema also develops (Sibon et al, 2004; Orakcioglu et al, 2005). The presence of </p><p>16 cytotoxic edema indicates the global ischemia injury that occurs at the time of SAH, as cytotoxic edema occurs largely because of failure of energy-dependent Na ion/K ion pumps. Using diffusion gradient MRI techniques, cellular swelling (edema) is noted to develop after a propagating wave of ischemia, which can be seen spreading throughout the ipsilateral and contralateral hemispheres. Using these MRI techniques a fall of the apparent diffusion gradient as early as 2 min. after the SAH have been shown to occur, confirming a global ischemia insult with its evolving global cytotoxic edema (Busch et al, 1998). It appears that the first phase of the biphasic response results in immediate brain edema. This gives rise to an increase in the ICP, which further reduces CBF, leading to further ischemia. As a result there is more damage to the BBB, which culminates in the initiation of the apoptotic cascades, which leads to further breakdown of the BBB, and the second phase of the biphasic response. It is the disruption of the endothelial cells due to cell death brought about by apoptosis that allows for the acute rise in both cerebral edema and ventricular volumes (Laszlo et at, 1995). This is in essence the foundation of the concept that brain edema contributes to the rise in ICP seen immediately after SAH (Hayashi et al, 1977). It is also believed to result in acute vasoconstriction, which combined with edema leads to further reduction in CSF, resulting in global ischemia injury (Doczi, 2001). What is of interest from a treatment standpoint is that experimentally reduction in brain edema as well as preservation of the BBB in SAH models using rats has been accomplished using caspase 3 inhibitors (z-VAD-FMK) (Park et al, 2004) and p53 inhibitors (Pifithrin) (Zhou et al, 2005). Claassen et al have also demonstrated that delayed global edema has been shown to be an independent predictor of death. The psychological problems experienced by SAH survivors are a well-known phenomenon. Even patients with a relatively benign SAH and postoperative course describe long-term memory and concentration problems. Some insight into the etiology of this phenomenon has been elucidated using SAH animal models, which have demonstrated profound hippocampal neuronal loss within a relatively short period of time following initiation of the SAH. Some studies have shown up to 30% loss. It is believed the loss of hippocampal neurons occurs secondary to global ischemia, which occurs at the</p><p>17 time of the SAH, which is probably exacerbated by disruption of the BBB and brain edema. Although some degree of global ischemia occurs in all patients who have a SAH, what is most perplexing is the grade 1 patient’s, who complain only of a headache, experience no syncope, and yet have a profound ischemic loss of neurons in the hippocampus. Park et al demonstrated in animal models that you could prevent some degree of this profound loss of hippocampal neurons by using various apoptotic inhibitors. Necrosis has been demonstrated in two major areas in SAH; these are in the vasculature and circumventricular regions. Some studies have demonstrated necrotic cells in the smooth muscle layer of your major vessels, where they are believed to contribute to vasospasm (Hughes and Schianchi, 1978; Ogihara et al, 2001). Cell culture studies have suggested that oxyhemoglobin may play a leading roe with regard to necrosis in the smooth muscle layer. What is not clear however, is whether the necrosis in the vessel wall contributes to vasospasm or is in fact the result of vasospasm. This is due to the fact that energy metabolites within a vessel undergoing vasospasm are significantly diminished (Yoshimoto et al, 1993). It has also been shown that necrosis occurs after SAH in the circumventricular regions, which contributes to fluid and electrolyte imbalance (Akpinar et al, 2005). The two areas, which appear to be especially vulnerable, are the subfornical organ and the organum vasculosum lamina terminalis. The subfornical organ (SFO) is situated on the ventral surface of the fornix, at the foramen of Monro, and is one of the circumentricular organs of the brain. The other organs are the area postrema in the brainstem and the organum vasculosum of the lamina terminalis (OVLT). The OVLT and SFO are both interconnected with the nucleus medianus; together these three structures are referred to as the AV3V region – the region anterior and ventral to the third ventricle. The AV3V region is very important in the regulation of fluid and electrolyte balance, by controlling thirst, sodium excretion, blood volume regulation, and vasopressin secretion. What is important to remember is both the SFO and OVLT are outside the BBB, consequently the neurons in these regions can respond to factors that are present in the systemic circulation. Some of the neurons in the SFO and OVLT are osmoreceptors, being sensitive to the osmotic pressure of the blood. These neurons project to the supraoptic nucleus and paraventricular nucleus to regulate </p><p>18 the activity of the vasopressin-secreting neurons. In addition, the neurons in the SFO and OVLT have receptors for many hormones that circulate in the blood but which do not cross the BBB, including angiotensin, atrial natriuretic hormone, endothelin and relaxin. Conclusion The apoptotic pathways believed to play a major role in SAH-induced apoptosis are the death receptor and TNF-α, p53, and the caspase-dependent cascades. The exact role of caspase-independent and mitochondrial cascades in SAH has not been fully elucidated. Experimentally several different inhibitors have been used such as caspase 3 and p53 inhibitors. However, to date, multiple level inhibitors, such as selectively blocking the limiting steps of the caspase-independent and –dependent cascades and the Bcl-2 family proteins, have not been used. This would inhibit all known apoptotic routes. All of these pathways however have the same result, the death of the cell. Cell death after SAH has important implications not only for vasospasm but also for the long-term sequelae of SAH. In the previous article on non-traumatic SAH, I discussed the benign nature of perimesencephalic bleeds. What is of interest is why perimesencephalic bleeds are not associated with similar sequelae. Presently the feeling is that because of the limited nature of these hemorrhages they are not considered a stress event that rises to the level to initiate the apoptotic cascade. It appears that EBI is a combination of physiologic insults to the brain, resulting in global ischemia, BBB breakdown, edema, and cellular death signaling. These changes occur acutely and chronically, although after 72 hours vasospasm becomes the main event. The consequences of EBI can be seen immediately and in the long term. From a treatment perspective, the mitigation of both the immediate and long term consequences of SAH is going to require further research into what pathways in human beings are responsible for the not uncommon devastating sequelae of SAH. Hopefully this research will lead to the development of therapeutic regimes, using either single or multiple inhibitors, which may alleviate, to a substantive degree, the tragic molecular pathologic consequences of SAH. </p><p>19 20</p>
Details
-
File Typepdf
-
Upload Time-
-
Content LanguagesEnglish
-
Upload UserAnonymous/Not logged-in
-
File Pages20 Page
-
File Size-