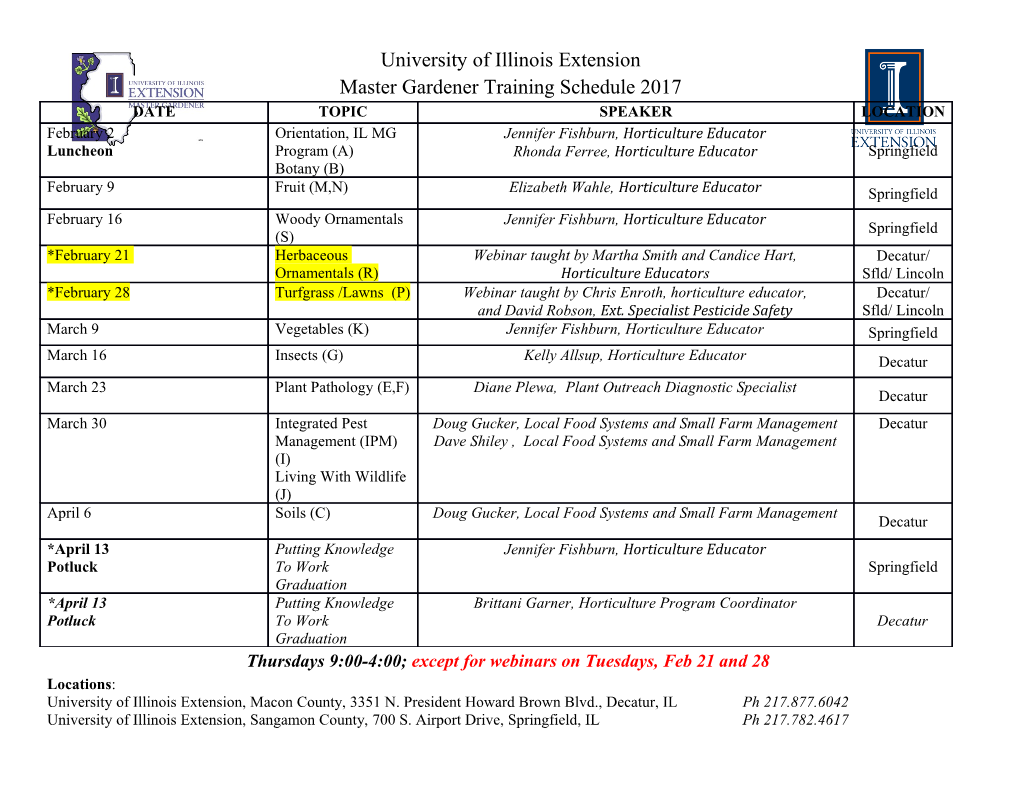
<p> 1</p><p>1 Neptunium and Manganese Biocycling in Nuclear Legacy Sediment</p><p>2 Systems</p><p>3 Clare L. Thorpe1, Katherine Morris1, Jonathan R. Lloyd1, Melissa A. Denecke2,</p><p>4 Kathleen A. Law2, Kathy Dardenne3, Christopher Boothman1, Pieter Bots1, Gareth T.</p><p>5 W. Law2*</p><p>6</p><p>7 1 Research Centre for Radwaste Disposal and Williamson Research Centre for Molecular</p><p>8 Environmental Science, School of Earth, Atmospheric and Environmental Sciences, The</p><p>9 University of Manchester, Manchester, M13 9PL, U.K.</p><p>10 2 Centre for Radiochemistry Research, School of Chemistry, The University of Manchester,</p><p>11 M13 9PL, U.K.</p><p>12 3 Karlsruhe Institute of Technology, Institut Fur Nukleare Entsorgung, D-76021 – Karlsruhe,</p><p>13 Germany. </p><p>14 2</p><p>15 *Corresponding author: Email: [email protected]; Tel: +44 (0)161 275 </p><p>16 1428Abstract </p><p>17 Understanding the behaviour of the highly radiotoxic, long half-life radionuclide neptunium</p><p>18 in the environment is important for the management of radioactively contaminated land and</p><p>19 the safe disposal of radioactive wastes. Recent studies have identified that microbial</p><p>20 reduction can reduce the mobility of neptunium via reduction of soluble Np(V) to poorly</p><p>21 soluble Np(IV), with coupling to both Mn- and Fe(III)- reduction implicated in neptunyl</p><p>22 reduction. To further explore these processes Mn(IV) as δMnO2 was added to sediment</p><p>23 microcosms to create a sediment microcosm experiment “poised” under Mn-reducing</p><p>24 conditions. Enhanced removal of Np(V) from solution occurred during Mn-reduction, and</p><p>25 parallel X-ray absorption spectroscopy (XAS) studies confirmed Np(V) reduction to Np(IV)</p><p>26 commensurate with microbially-mediated Mn-reduction. Molecular ecology analysis of the</p><p>27 XAS systems, which contained up to 0.2 mM Np showed no significant impact of elevated</p><p>28 Np concentrations on the microbial population. These results demonstrate the importance of</p><p>29 Mn cycling on Np biogeochemistry, and clearly highlight new pathways to reductive</p><p>30 immobilisation for this highly radiotoxic actinide. 3</p><p>31 Introduction</p><p>32 Internationally, deep geological disposal is being considered as the long-term management</p><p>33 and disposal option for higher activity radioactive wastes (HAW). A fundamental knowledge</p><p>34 of reactions between radionuclides and geomedia is essential to underpin the safety case for</p><p>35 geodisposal. Neptunium is a key risk-driving radionuclide in HAW due to its long half-life</p><p>36 (237Np t½ = 2.1 x 106 years), ingrowth from 241Am, high radiotoxicity, and relatively high</p><p>37 solubility as Np(V). Indeed, Np is potentially the most mobile transuranic species in</p><p>38 environments pertinant to deep geological disposal (e.g. Choppin and Stout, 1989; Kaszuba</p><p>39 and Runde, 1999; Lloyd et al., 2000; Choppin, 2007; Law et al., 2010); further, Np is a</p><p>40 persistant contaminant at or near nuclear sites (e.g. Cantrell, 2009; Morris et al., 2000;</p><p>41 Stamper et al., 2013).</p><p>42 Neptunium is redox active and it’s environmental mobility can be affected by the</p><p>43 biogeochmistry and redox conditions in the subsurface (Kaszuba and Runde, 1999; Lloyd et</p><p>44 al., 2002; Choppin, 2007; Law et al., 2010). Under oxidising conditions Np is stable in</p><p>+ 45 solution as the soluble neptunyl cation, NpO2 , whilst under anaerobic conditions Np can be</p><p>46 reduced to poorly soluble Np(IV) species (Kaszuba and Runde, 1999; Moyes et al., 2002;</p><p>47 Llorens et al., 2005; Law et al., 2010; Bach et al., 2014). In the subsurface, microbial</p><p>48 respiration can induce anaerobic conditions under which metals and radionuclides can be</p><p>49 reduced (Lloyd and Renshaw, 2005). The development of bioreducing conditions is</p><p>50 increasingly recognised as likely to be significant in the deep subsurface around a geological</p><p>51 disposal facility (Pedersen, 2000; Fredrickson and Balkwill, 2006; Rizoulis et al., 2012;</p><p>52 Williamson et al., 2013; Behrends et al., 2012), and is the basis for remediation of</p><p>53 contaminated land where problematic radionuclides (e.g. Tc and U) may be reduced either</p><p>54 enzymatically or indirectly via interactions with reduced species (e.g. Fe(II): Lloyd et al.,</p><p>55 2002; Lloyd, 2003; Gadd, 2010; Newsome et al., 2014). 4</p><p>56 The ability of microorganisms to enzymatically reduce Np(V) to Np(IV) has been</p><p>57 demonstrated in pure culture experiments (Lloyd et al., 2000; Icopini et al., 2007) although</p><p>58 some microorganisms are unable to facilitate enzymatic Np(V) reduction (Songkasiri et al.,</p><p>59 2002; Renshaw et al., 2005). In contrast, Gorman-Lewis et al., (2005) inferred potential non-</p><p>60 enzymatic reduction of Np(V) to explain trends in sorption / desorption experiments</p><p>61 conducted with Bacillus subtilis. Toxicity effects on selected metal-reducing bacteria are also</p><p>62 of interest as studies with indigenous microorganisms highlight the tolerence of</p><p>63 microorganisms to mM concentrations of Np (Law et al., 2010; Ams et al., 2013), whilst in</p><p>64 pure culture experiments no toxicity effects were observed at Np concentrations less than</p><p>65 2mM (Ruggiero et al., 2005). In sediment systems, reductive immobilisation of Np(V) to</p><p>66 Np(IV) has been observed during development of sediment anoxia with microbial metal</p><p>67 reduction implicated in the reaction and with indirect (abiotic) reduction by Fe(II) shown to</p><p>68 be possible (Law et al 2010).</p><p>69 Manganese is ubiquitous in soils and rock forming minerals and therefore, although Np</p><p>70 interactions with Mn minerals have been studied previously (Wilk et al., 2005), a deeper</p><p>71 understanding of Np(V) behaviour during early metal reduction (Mn- and Fe(III)-reduction)</p><p>72 is essential in understanding its environmental behaviour in both deep and shallow subsurface</p><p>73 environments. In addition, the potential importance of Mn in environmental actinide</p><p>74 chemistry is increasingly recognised with Mn linked to both Pu and U cycling (Powell et al.,</p><p>75 2006; Hu et al., 2010; Wang et al., 2013; Wang et al., 2014). Here we examine the behaviour</p><p>76 of Np in sediment systems amended with labile Mn(IV) (δMnO2) to allow microcosms to</p><p>77 develop a period of extended or “poised” Mn reduction (Lovley and Philips, 1988). As well</p><p>78 conducting experiments at low Np concentrations, we also collected XAS data from parallel</p><p>79 experiments run at higher concentrations of Np. This allowed assessment of Np speciation</p><p>80 and local-coordination under defined biogeochemical conditions. Finally, 16S rRNA gene 5</p><p>81 analysis was performed to assess the response of the indigenous microbial communities to</p><p>82 elevated Np concentrations.</p><p>83</p><p>84 Experimental Section </p><p>85 Safety</p><p>86 Neptunium (237Np) is a high radiotoxicity alpha-emitting radionuclide with beta/gamma</p><p>87 emitting progeny. Work can only be conducted by trained personnel in a certified, properly</p><p>88 equipped radiochemistry laboratory, following appropriate risk assessment. The possession</p><p>89 and use of radioactive materials is subject to statutory control.</p><p>90</p><p>91 Sample Collection</p><p>92 Sediments were collected from an area located ~ 2 km from the Sellafield reprocessing site in</p><p>93 Calder River Valley, Cumbria (Lat 54°26’30 N, Long 03°28’09 W). Sediments were</p><p>94 representative of the Quaternary unconsolidated alluvial flood-plain deposits that underlie the</p><p>95 Sellafield site (Law et al., 2010) and were collected in sterile containers, sealed, and stored at</p><p>96 4 ºC prior to use (< 1 month).</p><p>97</p><p>+ 98 Bioreduction Microcosms with Low NpO2 Concentrations </p><p>99 Sediment microcosms (10 ± 0.1 g Sellafield sediment, 100 ± 1 ml groundwater; in triplicate)</p><p>100 were prepared using a synthetic groundwater representative of the Sellafield region (Wilkins</p><p>101 et al., 2007) but with added nitrate and manganese (2 mM NaNO3, 2 mM δMnO2) and with a</p><p>102 total 0.17 mmols of bioavailable Fe(III) in the sediment. Sodium acetate was also added in</p><p>103 stoichiometric excess (10 mM) as an electron donor, the groundwater was sterilised</p><p>104 (autoclaved for 1 hour at 120 ºC), purged with filtered 80 % / 20 % N2 / CO2, and pH adjusted</p><p>105 to pH 7 (via drop-wise addition of 0.5 M HCl or 1M NaOH). Sediments and sterile 6</p><p>106 groundwater were then added to sterile 120 ml glass serum bottles (Wheaton Scientific, USA)</p><p>237 + 107 and sealed with butyl rubber stoppers using aseptic technique. Neptunium, as NpO2</p><p>108 (20 Bq ml-1; 3.2 µM; oxidation state verified by UV-Vis analysis) was then spiked into each</p><p>109 microcosm; thereafter, the microcosms were incubated anaerobically at 21 ºC in the dark for</p><p>110 38 days. Throughout the incubation, sediment slurry was periodically extracted using aseptic</p><p>111 technique, under an O2-free, Ar atmosphere. The sediment slurry was centrifuged (15,000 g;</p><p>112 10 minutes) to separate sediment and porewater samples and ~ 0.5 g of sediment was stored</p><p>113 at - 80 ºC for later microbiological characterisation.</p><p>114</p><p>115 Geochemical Analyses</p><p>116 During microcosm sampling, total dissolved Mn and Fe concentrations were measured with</p><p>117 standard UV-vis spectroscopy methods on a Jenway 6715 spectrophotometer (Lovley and</p><p>- 2- 118 Philips, 1987; Goto et al., 1997; Viollier et al., 2000). Aqueous NO3 , SO4 , ammonium and</p><p>119 acetate were measured by ion chromatography (Dionex ICS5000). Total bioavailable Fe(III)</p><p>120 and the proportion of extractable Fe(II) in the sediment was estimated by digestion of 0.1 g of</p><p>121 sediment in 5 ml of 0.5 N HCl for 60 minutes followed by the ferrozine assay, with and</p><p>122 without added hydroxylammonium chloride (Lovley and Phillips, 1987; Viollier et al., 2000).</p><p>123 The pH and Eh were measured with an Orion 420A digital meter and calibrated electrodes.</p><p>124 Standards were routinely used to check the reliability of all methods and typically, calibration</p><p>125 regressions had R2 ≥ 0.99. The elemental composition and bulk mineralogy of the sediment</p><p>126 were determined by XRF (Thermo ARL 9400) and XRD (Philips PW 1050). Total organic</p><p>127 carbon and total inorganic carbon were determined on a LECO CR-412 Carbon Analyser.</p><p>128 The total 237Np concentration in solution was measured by ICP-MS (Agilent 7500cx) using</p><p>129 232Th as the internal standard. </p><p>130 7</p><p>131 XAS Experiments</p><p>132 Experiments were prepared to allow direct determination of Np speciation and local</p><p>133 coordination environment in sediments under different geochemical conditions using X-ray</p><p>134 Absorption Spectroscopy (XAS). Here, the elevated concentration of Np required for direct</p><p>+ 135 spectroscopic characterisation (0.2 mM Np(V) as NpO2 in 0.07 M HCl) was added to</p><p>136 microcosms containing 1g of sediment and 10 ml of groundwater that were poised at oxic,</p><p>137 nitrate-, Mn-, Fe(III)-, and sulfate-reducing conditions, respectively. After Np(V) addition,</p><p>138 the microcosms were left to incubate for 1 week in the dark at 7 ºC prior to geochemical</p><p>139 sampling and subsequent freezing at – 80 ºC. Two additional Mn-reducing XAS systems</p><p>+ 140 were also established where sediments had 2 mM of δMnO2 added: (i) 0.2 mM NpO2 was</p><p>141 added to an oxic microcosm that was then left to progress to Mn-reducing conditions</p><p>142 (verified by the presence of Mn in porewaters and the absence of detectable 0.5 N extractable</p><p>143 Fe(II) in sediments) before freezing at -80 oC, and (ii) a parallel Mn-reducing microcosm</p><p>144 (again with no detectable 0.5 N extractable Fe(II) in sediments) that was sterilised by</p><p>+ 145 autoclaving (1 hour at 120 ºC) prior to the addition of 0.2 mM NpO2 , and which was frozen</p><p>146 at -80 oC 2 days after Np(V) addition. For XAS analysis, sediment samples were defrosted,</p><p>147 centrifuged, and ~ 0.5 g of sediment was packed (under anaerobic atmosphere if necessary)</p><p>148 into airtight sample containers which were then triple contained and frozen until analysis.</p><p>149 XAS analysis was conducted at the INE Beamline for Actinide Research at the ANKA</p><p>150 synchrotron, Karlsruhe, Germany. Neptunium LIII-edge spectra (17610 eV) were collected in</p><p>151 fluorescence mode by a 5 element solid-state Ge detector. Parallel K-edge measurements</p><p>152 from a Zr foil were recorded for energy calibration. XANES data were collected for all</p><p>153 samples and EXAFS data were collected for selected samples. Background subtraction, data</p><p>154 normalization, and fitting to EXAFS spectra were performed using the software packages 8</p><p>155 Athena and Artemis. The XANES edge-jump was tied to unity. Modeling of the EXAFS</p><p>156 data in k3 range was completed between 3 and 9.5 Ǻ-1.</p><p>157</p><p>158 Microbial Community Analysis</p><p>159 Samples from an oxic sediment, and a Mn-reducing sample were taken from both low Np</p><p>160 (20 Bq ml-1; 3.2 μM) and high Np (1.3 kBq ml-1; 0.2 mM) microcosms and analysis was</p><p>161 performed using PCR-based 16S rRNA gene analysis.</p><p>162</p><p>163 Ribosomal Intergenic Spacer Analysis</p><p>164 DNA was extracted from Np containing microcosm samples (200 µl) using a PowerSoil</p><p>165 DNA Isolation Kit (MO BIO Laboratories INC, USA). The 16S-23S rRNA intergenic spacer</p><p>166 region from the bacterial RNA operon was amplified as described previously using primers</p><p>167 ITSF and ITSReub (Cardinale et al., 2004). The amplified products were separated by</p><p>168 electrophoresis in tris-acetate-EDTA gel. The DNA was stained with ethidium bromide and</p><p>169 viewed under short-wave UV light. Positive microbial community changes identified by the</p><p>170 RISA justified further investigation by DNA sequencing of 16S rRNA gene clone libraries. </p><p>171 Amplification of 16S rRNA Gene Sequences</p><p>172 A fragment of the 16S rRNA gene (approximately 1490 b.p.) was amplified from samples</p><p>173 using the broad-specificity primers 8F (Eden et al., 1991) and 1492R (Lane et al., 1985). PCR</p><p>174 reactions were performed in thin-walled tubes using a BioRad iCycler (BioRad, UK). Takara</p><p>175 Ex Taq Polymerase (Millipore, UK) was used to amplify DNA from the sample extract. The</p><p>176 PCR amplification protocol used with the 8F and 1492R primers was: initial denaturation at</p><p>177 94 ºC for 4 minutes, melting at 94 ºC for 30 seconds, annealing at 50 ºC for 30 seconds,</p><p>178 elongation at 72 ºC for 3 minutes and 35 cycles, followed by a final extension step at 72 ºC 9</p><p>179 for 5 minutes. The purity of the amplified products was determined by electrophoresis in tris-</p><p>180 acetate-EDTA (TAE) gel. DNA was stained with ethidium bromide and viewed under short-</p><p>181 wave UV light using a BioRad Geldoc 2000 system (BioRad, UK). </p><p>182 Cloning</p><p>183 PCR products were purified using a QIAquick PCR purification kit (Qiagen, UK) and ligated</p><p>184 directly into a cloning vector containing topoisomerase I-charged vector arms (Agilent</p><p>185 Technologies, UK) prior to transformation into E. coli competent cells expressing Cre</p><p>186 recombinase (Agilent Technologies, UK). White transformants that grew on LB agar</p><p>187 containing ampicillin and X-Gal were screened for an insert using PCR. Primers were</p><p>188 complementary to the flanking regions of the PCR insertion site of the cloning vector. The</p><p>189 PCR method was: an initial denaturation at 94 ºC for 4 minutes, melting at 94 ºC for 30</p><p>190 seconds, annealing at 55 ºC for 30 seconds, extension at 72 ºC for 1 minutes and 35 cycles,</p><p>191 followed by a final extension step at 72 ºC for 5 minutes. The resulting PCR products were</p><p>192 purified using an ExoSap protocol, here 2 μl of ExoSap mix (0.058 μl Exonuclease I, 0.5 μl</p><p>193 Shrimp Alkaline Phosphatase and 1.442 μl H2O) was added to 5 μl of PCR product and</p><p>194 incubated at 37 ºC for 30 minutes followed by 80 ºC for 15 minutes.</p><p>195 DNA Sequencing and Phylogenetic Analysis</p><p>196 Nucleotide sequences were determined by the dideoxynucleotide method. An ABI Prism</p><p>197 BigDye Terminator Cycle Sequencing Kit was used in combination with an ABI Prism 877</p><p>198 Integrated Thermal Cycler and ABI Prism 377 DNA Sequencer (Perkin Elmer Applied</p><p>199 Biosystems, UK). Sequences (typically 900 base pairs in length) were analysed using</p><p>200 Mallard (Ashelford et al., 2006) to check for presence of chimeras or sequencing anomalies.</p><p>201 Operational taxonomic units (OTU) were determined at a 98 % sequence similarity level</p><p>202 using Mothur (Schloss et al., 2009). The individual OTU sequences were analysed using the 10</p><p>203 sequencing database of known 16S rRNA gene sequences provided on the Ribosomal</p><p>204 Database Project (Cole et al., 2009) to identify nearest neighbours.</p><p>205</p><p>206 Results and Discussion </p><p>207 Sediment Characteristics</p><p>208 The sediment was dominated by quartz, feldspars (albite and microcline), and sheet silicates</p><p>209 (muscovite and chlorite). The sediment had a high Si content (36.3 wt %) and contained Al</p><p>210 (6.77 %), Fe (3.71 %), K (2.67 %), Na (0.92 %), Mg (0.75 %), Ti (0.38 %), Ca (0.27 %), and</p><p>211 Mn (0.09 %). The total organic carbon content of the sediment was 0.69 % and total carbon</p><p>212 was 1.70 %. The concentration of 0.5 N HCl extractable Fe in the sediment was</p><p>213 17.1 ± 1.6 mmol kg-1 prior to incubation and the sediment pH was ~ 5.</p><p>214</p><p>215 Neptunium behaviour during progressive bioreduction </p><p>216 Manganese enriched (2 mM δMnO2) sediment microcosms amended with electron donor, and</p><p>217 a Mn enriched sterile control microcosm, were spiked with 3.2 µM Np(V) and incubated over</p><p>218 a 38 day period. Modelling of the initial groundwater chemistry in PHREEQC-2 (Specific</p><p>219 Ionic Theory (SIT) database) predicted that the speciation of the Np in solution would be</p><p>+ 220 predominantly NpO2 (see supporting information S1). In the sterile-control system, the pH</p><p>221 remained stable and bulk biogeochemical changes indicative of terminal electron acceptor</p><p>222 progression were not observed (Figure 1 A-F). A release of Mn (~0.2 mM) into the</p><p>223 groundwater of the sterile control occurred when the sediments were autoclaved (Figure 1C)</p><p>224 which is similar to past studies with this material (Thorpe et al., 2012). In the microbially-</p><p>225 active Mn rich microcosms, terminal electron accepting processes progressed in the order</p><p>- 2- 226 NO3 > Mn > Fe(III) > SO4 reduction as expected (Figure 1B-E). In all microcosms, the pH</p><p>- 227 remained circumneutral (Figure 1F), NO3 decreased to < 0.2 mM within 11 days, and 11</p><p>- 228 porewater Mn increased to between 0.05 – 0.1 mM after 9 days suggesting concomitant NO3</p><p>229 and Mn reduction in these systems (Figure 1B and C). Microbially-mediated Fe(III)</p><p>230 reduction was then evident after 17 days as indicated by 0.5 N HCl extractable Fe(II)</p><p>231 ingrowth to sediments. Importantly, in this system Mn reduction (indicated by Mn(II) in</p><p>232 porewaters) occurred independently of any measurable Fe(III) reduction (indicated by a lack</p><p>233 of Fe(II) ingrowth to sediments) across three time-points (days 7 to 11; Figure 1). The</p><p>234 addition of 2 mM δMnO2 to sediments then allowed an extended Mn-reducing ‘period’,</p><p>235 distinguished from the Fe(III)-reduction, so that Np-behaviour could be tracked throughout</p><p>236 the stages of early metal reduction. In the sterile control the added Np(V) was partially sorbed</p><p>237 to the sediment, with ~ 22 % removed from solution after 7 days (Figure 1A). Thereafter, the</p><p>238 concentration of Np in solution remained stable. Neptunyl sorption has been observed to a</p><p>239 similar extent as in earlier studies using comparable sediment systems (Law et al., 2010) and</p><p>240 has been attributed to sorption to negatively charged mineral surfaces (e.g. Fe(III)- or Mn-</p><p>241 bearing minerals: Combes et al., 1992; Nakata et al., 2002; Arai et al., 2007; Müller et al.,</p><p>242 2015; Wilk et al., 2005). In the microbially active microcosms prior to the onset of Mn</p><p>243 ingrowth to porewaters (0 - 7 days), 43.0 ± 1.9 % of the added Np was removed from the</p><p>244 groundwater (Figure 1A). By day 11 where Mn-reducing conditions had developed, 86.0 ±</p><p>245 4.9 % of the added Np had been removed from solution. By the end of the experiment,</p><p>2- 246 following Fe(III) and subsequent SO4 reduction at 38 days, 96.1 ± 0.5 % of the added Np</p><p>247 was removed to the sediment. Enhanced removal of Np in active systems, compared to the</p><p>248 sterile control, as observed in the first 7 days and prior to the onset of Mn reduction, could be</p><p>249 attributed to either reduced surface reactivity in autoclaved sediments and / or enhanced Np</p><p>250 sorption in the system with microbial cells present (Gorman-Lewis et al., 2005; Ams et al.,</p><p>251 2013). Results then show a clear relationship between Np(V) removal from solution and Mn</p><p>252 reduction and confirms that Np(V) is significantly removed from groundwater under Mn- 12</p><p>253 reducing conditions. It remains unclear in these low concentration Np microcosm studies</p><p>254 whether Np(V) removal is linked to microbial metabolism or the result of abiotic reaction</p><p>255 with reduced Mn minerals produced by microbes. The formation of Np-carbonatohydroxo</p><p>256 complexes has been shown to increase the solubility of Np(IV) (Kitamura and Kohara, 2002;</p><p>257 Kim et al., 2010). However, in these systems under end-point sulfate reducing conditions (pE</p><p>258 -4), and taking into account the increase in inorganic carbon expected from acetate utilisation</p><p>259 (2 mM), solution modelling in PHREEQC-2 (SIT database) predicted that Np would be</p><p>260 speciated as Np(OH)4 (see supporting information S2). </p><p>261</p><p>262 Neptunium LIII-edge XAS Experiments</p><p>263 To assess the speciation of Np in sediment microcosms under different biogeochemical</p><p>264 conditions, select samples were run at the elevated concentrations required for XAS analysis</p><p>265 (oxic, nitrate-, Mn-, Fe(III)-, sulfate-, progressive Mn- and sterilised Mn- reducing</p><p>- 266 microcosms). The XANES of Np in the sterile, oxic control sediment and in the NO3</p><p>267 reducing sediment both showed a Np(V)-like spectra displaying the characteristic multiple</p><p>268 scattering resonance structure at the high energy flank of the white line resulting from</p><p>269 scattering along the axial oxygen atoms of the linear neptunyl moiety (Figure 2; Moyes et al.,</p><p>270 2002; Denecke et al., 2005). Removal of Np from solution in both the oxic and nitrate</p><p>271 reducing systems at circumneutral pH is occurring and is likely due to Np(V) sorption to Fe</p><p>272 or Mn mineral surfaces (e.g. Combes et al., 1992; Nakata et al., 2002; Wilk et al., 2005; Arai</p><p>273 et al., 2007; Law et al., 2010; Müller et al., 2015). By contrast, the XANES spectra for the</p><p>2- 274 progressive Mn-reducing microcosm, and the poised Mn-, Fe(III)-, and SO4 - reducing</p><p>275 systems showed Np(IV)-like features with a loss of the multiple scattering resonance</p><p>276 structure due to the loss of the two neptunyl dioxygenyl oxygen backscatterers (Figure 2).</p><p>277 Here, the enhanced removal of Np from solution compared to the oxic or nitrate-reducing 13</p><p>278 systems is attributed to reductive precipitation of Np(V) to Np(IV) (Law et al., 2010). In the</p><p>279 absence of defined (matrix-matched) standards for these complex systems, linear</p><p>280 combination fitting of the microbially-active Mn-reducing systems (both progressively</p><p>281 reduced, and poised), using the oxic sediment and the sulfate-reducing sediment as end-</p><p>282 members, indicated that Np(IV) was indeed the dominant oxidation state in both systems</p><p>283 (>90 % Np(IV)) (Ravel et al., 2005). In contrast, the XANES spectra for the sterile Mn-</p><p>284 reduced microcosm was Np(V) like (> 90 % Np(V)). Interestingly, the presence of significant</p><p>285 Np(IV) (~90 %) in the microbially-active Mn-reducing systems and dominant Np(V) (~ 90</p><p>286 %) in the sterile Mn-reducing sediments suggests that microbial reduction of Np(V) to</p><p>287 Np(IV) is significant in reductive immobilisation of Np(V). Any artefacts associated with</p><p>288 mineral reactivity and reducing capacity of the sterile Mn-reducing sediment resulting from</p><p>289 autoclaving cannot however be ruled out here, but the significant change in Np(V) reduction</p><p>290 between the microbially active and sterile sediments suggests enzymatic processes are likely</p><p>291 to play a role in controlling Np(V) reductive immobilisation. These observations on sterile</p><p>292 Mn-reducing sediments differ from those observed in a sterilised Fe(III)-reducing sediment</p><p>293 reacted with Np(V), which facilitated Np(V) reduction (Law et al., 2010).</p><p>2- 294 EXAFS data were also collected from the sterile oxic and microbially-active Mn-, and SO4 -</p><p>295 reducing samples. The k3-weighted EXAFS spectra and their Fourier transform spectra are</p><p>296 shown together with the corresponding best model fits (Figure 3; Table 1). The best fit to the</p><p>297 sterilised oxic control sample was a Np(V)-like coordination environment with two axial</p><p>298 oxygen backscatterers at 1.86 Å and four equatorial oxygen backscatterers at 2.45 Å (Table 1,</p><p>299 Figure 3). The distances for both axial and equatorial oxygen backscatterers are within the</p><p>300 range reported for Np(V) (1.82 – 1.88 Å; Combes et al., 1992; Moyes et al., 2002; Denecke et</p><p>301 al., 2005; Arai et al., 2007; Herberling et al., 2007; Law et al., 2010). The statistical</p><p>302 relevance of additional shells, containing Fe, Mn, or Np, was assessed using an F-test (Ravel 14</p><p>303 et al., 2005) and it was found that no significant improvement to the model fit could be</p><p>304 achieved. The sulfate reducing sample was modelled using a Np(IV) like coordination</p><p>305 environment with eight oxygen backscatterers at a distance of 2.34 Å (Table 1, Figure 3;</p><p>306 Llorens et al., 2005; Law et al., 2010). In agreement with the linear combination fitting, the</p><p>307 best fit for the microbially active Mn-reducing sample was a Np(IV) like coordination</p><p>308 environment, like the sulfate reducing system, with eight oxygen backscatterers at a distance</p><p>309 of 2.31 Å. As with the oxic sediment sample, the addition of a second shell of Fe, Np or Mn</p><p>310 did not significantly improve the fit when using the F test as a measure of validity. Finally, it</p><p>311 is noteworthy that in the samples where significant Np(IV) was present and where EXAFS</p><p>312 was possible (the Mn-reducing sediment and sulfate-reducing microbially active sediments</p><p>313 (Figure 3)), there was no evidence for a Np – O – Np interaction of the type that would be</p><p>314 expected for nano-particulate NpO2, which has recently been observed in environmentally</p><p>315 relevant systems (Husar et al., 2015). These observations are in agreement with past work in</p><p>316 sediment systems (Law et al., 2010) which do not show significant evidence for a Np – O –</p><p>317 Np interaction. </p><p>318</p><p>319 Microbial community analysis</p><p>320 Analysis of the microbial community in the Mn-reducing systems with low (3.2 µM) and</p><p>321 high (0.2 mM) Np content was performed to provide insight into the toxicity of Np(V) in</p><p>322 these systems. The biogeochemical trajectory was similar in sediments with both low and</p><p>323 high concentrations of Np, suggesting that the toxicity of Np in these systems was not</p><p>324 significant. These observations were supported by the RISA results, which were similar</p><p>325 across both low and high concentration Np amended metal-reducing samples, and clone</p><p>326 libraries for the same samples, again confirming broadly similar communities (Figure 4).</p><p>327 Both high and low concentration Np experiments did however show a decrease in 15</p><p>328 biodiversity compared to the oxic sediment sample (clone libraries from the oxic sediment</p><p>329 showed 34 operational taxonomic units (OTUs) from 71 clones whereas the biostimulated</p><p>330 samples showed 7 OTUs from 92 clones in the low Np sample and 12 OTUs from 74 clones</p><p>331 in the high Np sample). The clone libraries of both low and high Np concentration Mn-</p><p>332 reducing samples were dominated (> 50 %) by members of the class Bacillus, including</p><p>333 known denitrifying species consistent with the microcosms being primed with 2 mM nitrate</p><p>334 prior to Mn reduction. </p><p>335</p><p>336 Conclusions</p><p>337 Overall these data show that microbially-mediated Mn reduction can lead to reductive</p><p>338 immobilisation of Np(V) to Np(IV). The addition of bioavailable δMnO2 provides a useful</p><p>339 approach for prolonging microbial Mn reduction and allowing discrimination between the</p><p>340 impacts of microbially-mediated Mn and Fe(III) reduction on radionuclide biogeochemistry.</p><p>341 Removal of Np during Mn reduction was further maintained during Fe(III) and sulfate</p><p>342 reduction and near complete removal of Np from solution had occurred by the onset of</p><p>343 sulfate reduction. XANES data confirmed reduction to Np(IV) when Np(V) was exposed to</p><p>344 microbially active Mn-, Fe(III)-, and sulfate-reducing sediments. Thermodynamically, Mn</p><p>345 reduction is a more favourable process than Fe(III) reduction and so is likely to occur prior to</p><p>346 Fe(III) reduction in subsurface environments where electron donor is limited. Although not</p><p>347 conclusive these results imply that Np(V) reduction in these systems occurs in the presence of</p><p>348 a viable microbial population rather than abiotically, through reaction with reduced Mn</p><p>349 bearing minerals. Reduction of Np(V) by metal-reducing bacteria may provide an additional</p><p>350 mechanism for Np(V) removal from groundwater ahead of the development of robust Fe(III)-</p><p>351 reducing conditions. Results show the importance of subsurface microbial manganese 16</p><p>352 cycling on the speciation of neptunium. These data have relevance to the fundamental</p><p>353 understanding of Np behaviour in the shallow and deep subsurface.</p><p>354</p><p>355 Acknowledgments </p><p>356 This work has been supported by the Natural Environmental Research Council grants</p><p>357 NE/H007768/1 and NE/L000547/1, an EPSRC University of Manchester / Sellafield Ltd.</p><p>358 KTA award for which we acknowledge Nick Atherton and the Sellafield Land Quality Team,</p><p>359 and STFC award ST/K001787/1. We thank Paul Lythgoe and Alastair Bewsher at The</p><p>360 University of Manchester, and Jörg Rothe (KIT-INE) for help with data acquisition. </p><p>361 Beamtime was obtained from the ANKA Lightsource (proposal number ANS-85) with</p><p>362 support from the EU Actinet Programme.</p><p>363</p><p>364 References</p><p>365 Ams, D.A., Swanson, J.S., Szymanowski, J.E.S., Fein, J.B., Richmann, M., Reed, D.T.</p><p>366 (2013). The effect of high ionic strength on neptunium(V) adsorption to a halophilic</p><p>367 bacterium. Geochim. Cosmochim. Acta, 110, 45-57.</p><p>368 Arai, Y., Moran, P. B., Honeyman, B.D., Davis, J.A. (2007). In situ spectroscopic evidence</p><p>369 for neptunium(V)-carbonate inner-sphere and outer-sphere ternary surface complexes</p><p>370 on hematite surfaces. Environ. Sci. Technol., 41, 3940-3944.</p><p>371 Ashelford, K.E., Chuzhanova, N.A., Fry, J.C., Jones, A.J., Weightman, A.J. (2006). New</p><p>372 screening software shows that most recent large 16S rRNA gene clone libraries</p><p>373 contain chimeras. Appl. Environ. Microbiol., 72, 5734–5741.</p><p>374 Bach, D., Christiansen, B.C., Schild, D., Geckeis, H. (2014). TEM study of green rust sodium</p><p>+ 375 sulfate (GRNa.SO4) interacted with neptunyl ions (NpO2 ). Radiochim. Acta, 102(4),</p><p>376 279-290. 17</p><p>377 Behrends, T., Krawczyk-Bärsch, E., Arnold, T. (2012). Implementation of microbial processes</p><p>378 in the performance assessment of spent nuclear fuel repositories. Appl. Geochem., 27,</p><p>379 453-462.</p><p>380 Cantrell, K.J. (2009). Transuranic contamination in sediment and groundwater at the U.S.</p><p>381 DOE Hanford site. US Department of Energy Report: PNNL-18640. Available at:</p><p>382 http://www.pnl.gov/main/publications/external/technical_reports/PNNL-18640.pdf</p><p>383 Cardinale, M., Brusetti, L., Quatrini, P., Borin, S., Puglia, A.M., Rizzi, A., Zanardini, E.,</p><p>384 Sorlini, C., Corselli, C., Daffonchio, D. (2004). Comparison of different primer sets</p><p>385 for use in automated ribosomal intergenic spacer analysis of complex bacterial</p><p>386 communities. Appl. Environ. Microbiol., 70, 6147–6156.</p><p>387 Choppin, G.R., Stout, B.E. (1989). Actinide behaviour in natural waters. Sci. Tot. Environ.,</p><p>388 83, 203-216. </p><p>389 Choppin, G.R. (2007). Actinide speciation in the environment. J. Radioanal. and Nucl.</p><p>390 Chem., 273, 695–703.</p><p>391 Cole, J.R., Wang, Q., Cardenas, E., Fish, J., Chai, B., Farris, R.J., Kulam-Syed-Mohideen,</p><p>392 A.S., McGarrell, D.M., Marsh, T., Garrity, G.M., Tiedje, J.M. (2009). The Ribosomal</p><p>393 Database Project: improved alignments and new tools for rRNA analysis. Nucleic</p><p>394 Acids Res., 37, 141-145.</p><p>395 Combes, J.M., Chisholm-Brause, C.J., Brown, G.E., Parks, G.A., Conradson, S.D., Eller,</p><p>396 P.G., Triay, I.R., Hobart, D.E., Miejer, A. (1992). EXAFS spectroscopic study of</p><p>397 neptunium(V) sorption at the α-iron hydroxide oxide α-FeOOH/water interface.</p><p>398 Environ. Sci. Technol., 26(2), 376-382.</p><p>399 Denecke, M.A., Dardenne, K., Marquardt, C.M. (2005). Np(IV)/Np(V) valence</p><p>400 determinations from Np L3-edge XANES/EXAFS. Talanta, 65, 1008–1014. 18</p><p>401 Eden, P.E., Schmidt, T.M., Blakemore, R.P., Pace, N.R. (1991). Phylogenetic analysis of</p><p>402 Aquaspirillum magnetotacticum using polymerase chain reaction-amplified 16S</p><p>403 rRNA-specific DNA. Int. J. Syst. Bacteriol., 41, 324-325.</p><p>404 Fredrickson, J.K., Balkwill, D.L. (2006). Geomicrobial processes and biodiversity in the deep</p><p>405 terrestrial subsurface. Geomicro. J., 23, 345-356.</p><p>406 Gadd, G.M. (2010). Metals, minerals and microbes: geomicrobiology and bioremediation.</p><p>407 SGM prize lecture. Microbiol., 156, 609-643.</p><p>408 Gorman-Lewis, D., Fein, J.B., Soderholm, L., Jensen, M.P., Chiang, M.H. (2005).</p><p>409 Experimental study of neptunyl adsorption onto Bacillus subtilis. Geochim.</p><p>410 Cosmochim. Acta, 69, 4837-4844.</p><p>411 Goto, K., Taguchi, S., Fukue, Y., Ohta, K., Watanabe, H. (1997). Spectrophotometric</p><p>412 determination of manganese with 1-(2-pyridylazo)-2-naphthol and a non-ionic</p><p>413 surfactant. Talanta, 24, 752-753.</p><p>414 Herberling, F., Denecke, M.A., Bosbach, D. (2008). Neptunium(V) co-precipitation with</p><p>415 calcite. Environ. Sci. Technol., 42(2), 471-476.</p><p>416 Hu, Y., Schwaiger, L.K., Booth, C.H., Kukkadapu, R.K., Cristiano, E., Kaplan, D., Nitsche,</p><p>417 H. (2010) Molecular interactions of plutonium(VI) with synthetic manganese-</p><p>418 substituted goethite. Radiochim. Acta, 98, 655–663.</p><p>419 1. Husar, R., Hübner, R., Hennig, C., Martin, P.M., Chollet, M., Weiss, S.,</p><p>420 Stumpf, T., Zänker, H., Ikeda-Ohno, A. (2015) Intrinsic formation of nanocrystalline</p><p>421 neptunium dioxide under neutral aqueous conditions relevant to deep geological</p><p>422 repositories. Chem. Commun., 51, 1301-1304.</p><p>423 Icopini, G.A., Boukhalfa, H., Neu, M.P. (2007). Biological reduction of Np(V) and Np(V)</p><p>424 citrate by metal-reducing bacteria. Environ. Sci. Technol., 41(8), 2764-2769. 19</p><p>425 Kaszuba, J.P., Runde, W.H. (1999). The aqueous geochemistry of neptunium: Dynamic</p><p>426 control of soluble concentrations with applications to nuclear waste disposal. Environ.</p><p>427 Sci. Technol., 33, 4427-4433.</p><p>428 Kim, B.Y., Oh, J.Y., Baik, M.H., Yun, J.I. (2010) Effect of carbonate on the solubility of</p><p>429 neptunium in natural granitic groundwater. Nuc. Eng. Technol., 42 (5), 552-561. </p><p>430 Kitamura, A., Kohara, Y., (2002) Solubility of neptunium(IV) in carbonate media. J. Nuc.</p><p>431 Sci. Technol., 3, 294-297. </p><p>432 Lane, D.J., Pace, B., Olsen, G.J., Stahl, D.A., Sogin, M.L., Pace, N.R. (1985). Rapid</p><p>433 determination of 16S ribosomal-RNA sequences for phylogenetic analysis. P. Nat.</p><p>434 Acad. Sci., 82, 6955-6959.</p><p>435 Law, G. T. W., Geissler, A., Lloyd, J.R., Livens, F.R., Boothman, C., Begg, J.D.C., Denecke,</p><p>436 M.A., Rothe, J., Dardenne, K., Burke, I.T., Charnock, J.M., Morris, K. (2010).</p><p>437 Geomicrobiological redox cycling of the transuranic element neptunium. Environ.</p><p>438 Sci. Technol., 44, 8924-8929. </p><p>439 Llorens, I., Den Auwer, C., Moisy, P., Ansoborlo, E., Vidaud, C., Funke, H. (2005).</p><p>440 Neptunium uptake by serum transferrin. FEBS Journal, 272, 1739-1744.</p><p>441 Lloyd, J.R., Yong, P., Macaskie, L.E. (2000). Biological reduction and removal of Np(V) by</p><p>442 two microorganisms. Environ. Sci. Technol., 34, 1297-1301.</p><p>443 Lloyd, J.R., Chesnes, J., Glasauer, S., Bunker, D.J., Livens, F.R., Lovley, D.R. (2002).</p><p>444 Reduction of actinides and fission products by Fe(III)-reducing bacteria.</p><p>445 Geomicrobiol. J., 19, 103-120. </p><p>446 Lloyd, J.R. (2003). Microbial reduction of metals and radionuclides. FEMS Microbiol. Rev.,</p><p>447 27, 411-425. 20</p><p>448 Lloyd, J.R., Renshaw, J.C. (2005). Bioremediation of radioactive waste: radionuclide-</p><p>449 microbe interactions in laboratory and field-scale studies. Curr. Opin. Biotechnol., 16,</p><p>450 254-260.</p><p>451 Lovley, D. R., Phillips, E. J. P. (1988). Manganese inhibition of microbial iron reduction in</p><p>452 anaerobic sediments. Geomicrobiol. J., 6, 145-155.</p><p>453 Lovley, D.R., Phillips, E.J.P. (1987). Rapid assay for microbially reducible ferric iron in</p><p>454 aquatic sediments. Appl. Environ. Microbiol., 53, 1536-1540.</p><p>455 Morris, K., Butterworth, J.C., Livens, F.R. (2000). Evidence for the remobilization of</p><p>456 Sellafield waste radionuclides in an intertidal salt marsh, West Cumbria, UK. Estuar.</p><p>457 Coast. Shelf S., 51, 613-625.</p><p>458 Moyes, L.N., Jones, M.J., Reed, W.A., Livens, F.R., Charnock, J.M., Mosselmans, J.F.W.,</p><p>459 Hennig, C., Vaughan, D.J., Pattrick, R.A.D. (2002). An X-ray absorption</p><p>460 spectroscopy study of neptunium(V) reactions with mackinawite (FeS). Environ. Sci.</p><p>461 Technol., 36, 179-183.</p><p>462 Müller, K., Gröschel, A., Rossberg, A., Bok, F., Franzen, C., Brendler, V., Foerstendorf, H.</p><p>463 (2015). In situ spectroscopic identification of neptunium(V) inner-sphere complexes</p><p>464 on the hematite water interface. Environ. Sci. Technol., 49, 2560-2567. </p><p>465 Nakata, K., Nagasaki, S., Tanaka, S., Sakamoto, Y., Tanaka, T., Ogawa, H. (2002). Sorption</p><p>466 and reduction of neptunium(V) on the surface of iron oxides. Radiochim. Acta, 90(9-</p><p>467 11), 665-669. </p><p>468 Newsome, L., Morris, K., Lloyd, J.R. (2014). The biogeochemistry and bioremediation of</p><p>469 uranium and other priority radionuclides. Chem. Geo., 363, 164-184.</p><p>470 Pedersen, K. (2000). Exploration of deep intraterrestrial microbial life: current perspectives.</p><p>471 FEMS Microbiol. Lett., 185, 9-16. 21</p><p>472 Powell, B.A., Duff, M.C., Kaplan, D.I., Field, R.A., Newville, M., Hunter, D.B., Bertsh,</p><p>473 P.M., Coates, J.T., Eng, P., Rivers, M.L., Serkiz, S.M., Sutton, S.R., Triay, I.R.,</p><p>474 Vaniman, D.T. (2006). Plutonium oxidation and subsequent reduction by Mn(IV)</p><p>475 minerals in Yucca Mountain tuff. Environ. Sci. Technol., 140(11), 3508-3514. </p><p>476 Ravel, B., Newville, M. (2005). ATHENA, ARTEMIS, HEPHAESTUS: Data analysis for X-</p><p>477 Ray absorption spectroscopy using IFEFFIT. J. Synchro. Rad., 12, 537−541. </p><p>478 Renshaw, J.C., Butchins, L.J.C., Livens, F.R., May, I., Charnock, J.M., Lloyd, J.R. (2005).</p><p>479 Bioreduction of uranium: Environmental implications of a pentavalent intermediate.</p><p>480 Environ. Sci. Technol., 39, 5657-5660.</p><p>481 Rizoulis, A., Steele, H.M., Morris, K., Lloyd, J.R. (2012). The potential impact of microbial</p><p>482 metabolism during the geodisposal of intermediate level waste. Min. Mag., 76, 3261-</p><p>483 3270.</p><p>484 Ruggiero, C.E., Boukhalfa, H., Forsythe, J.H., Lack, J.G., Hersman, L.E., Neu, M.P. (2005).</p><p>485 Actinide and metal toxicity to prospective bioremediation bacteria. Environ.</p><p>486 Microbiol., 7, 88-97.</p><p>487 Stamper, A., McKinlay, C., Coughlin, D., Laws, F. (2013). Groundwater Annual Report</p><p>488 2012. Sellafield Ltd, Technical report: LQTD000032</p><p>489 Schloss, P.D., Westcott, S.L., Ryabin, T., Hall, J.R., Hartmann, M., Hollister, E.B.,</p><p>490 Lesniewski, R.A., Oakley, B.B., Parks, D.H., Robinson, C.J., Sahl, J.W., Stres, B.,</p><p>491 Thallinger, G.G., Van Horn, D.J., Weber, C.F. (2009). Introducing mothur: Open-</p><p>492 source, platform-independent, community-supported software for describing and</p><p>493 comparing microbial communities. Appl. Environ. Microbiol., 75, 7537-7541. </p><p>494 Songkasiri, W., Reed, D.T., Rittmann, B.E. (2002). Bio-sorption of neptunium(V) by</p><p>495 Pseudomonas fluorescens. Radiochim. Acta, 90, 785-789. 22</p><p>496 Thorpe, C.L., Law, G.T.W., Boothman, C., Lloyd, J.R., Burke, I.T., Morris, K. (2012).</p><p>497 Synergistic effect of high nitrate concentrations on sediment bioreduction.</p><p>498 Geomicrobiol. J., 29, 484-493.</p><p>499 Viollier, E., Inglett, P.W., Hunter, K., Roychoudhury, A.N., Van Cappellen, P. (2000). The</p><p>500 ferrozine method revisited: Fe(II)/Fe(III) determination in natural waters. Appl.</p><p>501 Geochem.,15, 785-790.</p><p>502 Wang, Z., Lee, S.W., Kapoor, P., Tebo, B.M. and Giammar, D.E. (2013). Uraninite oxidation</p><p>503 and dissolution induced by manganese oxide: A redox reaction between two insoluble</p><p>504 minerals. Geochim. Cosmochim. Acta, 100(1), 24-40.</p><p>505 Wang, Z., Xiong, W., Tebo, B.M. and Giammar, D.E. (2014). Oxidative UO2 dissolution</p><p>506 induced by soluble Mn(III). Environ. Sci. Technol., 48 (1), 289-298.</p><p>507 Wilk, P.A., Shaughnessy, D.A., Wilson, R.E., Nitsche, H. (2005). Interfacial interactions</p><p>508 between Np(V) and manganese oxide minerals manganite and hausmannite. Environ.</p><p>509 Sci. Technol., 39, 2608-2615.</p><p>510 Wilkins, M.J., Livens, F.R., Vaughan, D.J., Beadle, I., Lloyd, J.R. (2007). The influence of</p><p>511 microbial redox cycling on radionuclide mobility in the subsurface at a low-level</p><p>512 radioactive waste storage site. Geobiol., 5, 293-301.</p><p>513 Williamson, A.J., Morris, K., Shaw, S., Byrne, J.M., Boothman, C., Lloyd, J.R. (2013).</p><p>514 Microbial reduction of Fe(III) under alkaline conditions relevant to geological</p><p>515 disposal. Appl. Environ. Microbiol., 79(11), 3320-3326. 23</p><p>516 Figure Legends</p><p>517</p><p>- 518 Figure 1. Microcosm incubation time-series data. (A) Np in porewaters, (B) NO3 in</p><p>519 porewaters, (C) Mn in porewaters, (D) 0.5 N HCl % extractable sedimentary Fe as Fe(II), (E)</p><p>2- 520 SO4 in porewaters. ■ = microbially active microcosms; ● = sterile control microcosms.</p><p>521 Initial pH in all microcosms was ~ 7. Error bars represent 1σ experimental uncertainty from</p><p>522 triplicate microcosm experiments (where not visible error bars are within symbol size).</p><p>523</p><p>524 Figure 2. Np LIII–edge XANES spectra for Np amended sediments under different biological</p><p>525 conditions. Spectra from oxic sediment, nitrate-reducing sediment and sterilised Mn-reducing</p><p>526 sediment samples show the Np(V) like multiple scattering resonance structure resulting from</p><p>527 high energy scattering along the axial oxygen atoms of the linear neptunyl moiety. Spectra</p><p>528 from Mn-, Fe(III)-, sulfate- and progressive Mn-reducing sediment samples do not contain</p><p>529 this feature and are therefore more typical of Np(IV) XANES. </p><p>530</p><p>531 Figure 3. EXAFS spectra and Fourier transforms (uncorrected for phase shift of</p><p>532 backscattering atoms) for Np on sediments under different geochemical conditions. From top</p><p>533 to bottom: oxic, Mn- and sulfate-reducing conditions. Black lines are k3-weighted data and</p><p>534 grey lines are the best model fits to the data.</p><p>535</p><p>536 Figure 4. Microbial community analysis of: (A) oxic sediment at 0 days; (B) Mn-reducing</p><p>537 sediment amended with 3.2 µM 237Np; (C) Mn-reducing sediment amended with 0.2 mM</p><p>538 237Np. 24</p><p>539 Table 1 540 EXAFS modelling of Np LIII edge spectra for Np associated with sediments under different 541 biogeochemical conditions. </p><p>2 2 2 Path Type CN R (Ǻ) σ (Ǻ ) Xv R</p><p>Oxic 1 O 2 1.86 0.006 59.8 0.0079 2 O 4 2.45 0.021 Mn 1 O 8 2.31 0.014 217. 0.0130 Red 3</p><p>Sul 1 O 8 2.34 0.012 98.9 0.0227 Red 542 CN is the coordination number, R is the interatomic distance, σ2 is the Debye-Waller factor 2 2 543 (Ǻ ), Xv reduced chi square value and R is the least squares residual and is a measure of the 544 overall goodness of fit. 545</p><p>546</p><p>547</p><p>548</p><p>549 25</p><p>550 Figure 1</p><p>551</p><p>552 26</p><p>553</p><p>554</p><p>555</p><p>556</p><p>557</p><p>558</p><p>559</p><p>560</p><p>561</p><p>562</p><p>563</p><p>564</p><p>565 Figure 2 27</p><p>566</p><p>567</p><p>568</p><p>569</p><p>570</p><p>571</p><p>572</p><p>573</p><p>574</p><p>575</p><p>576</p><p>577</p><p>578</p><p>579 28</p><p>580</p><p>581 Figure 3</p><p>582</p><p>583</p><p>584</p><p>585</p><p>586</p><p>587</p><p>588</p><p>589</p><p>590</p><p>591</p><p>592</p><p>593 Figure 4 29</p><p>594</p><p>595</p><p>596</p>
Details
-
File Typepdf
-
Upload Time-
-
Content LanguagesEnglish
-
Upload UserAnonymous/Not logged-in
-
File Pages29 Page
-
File Size-