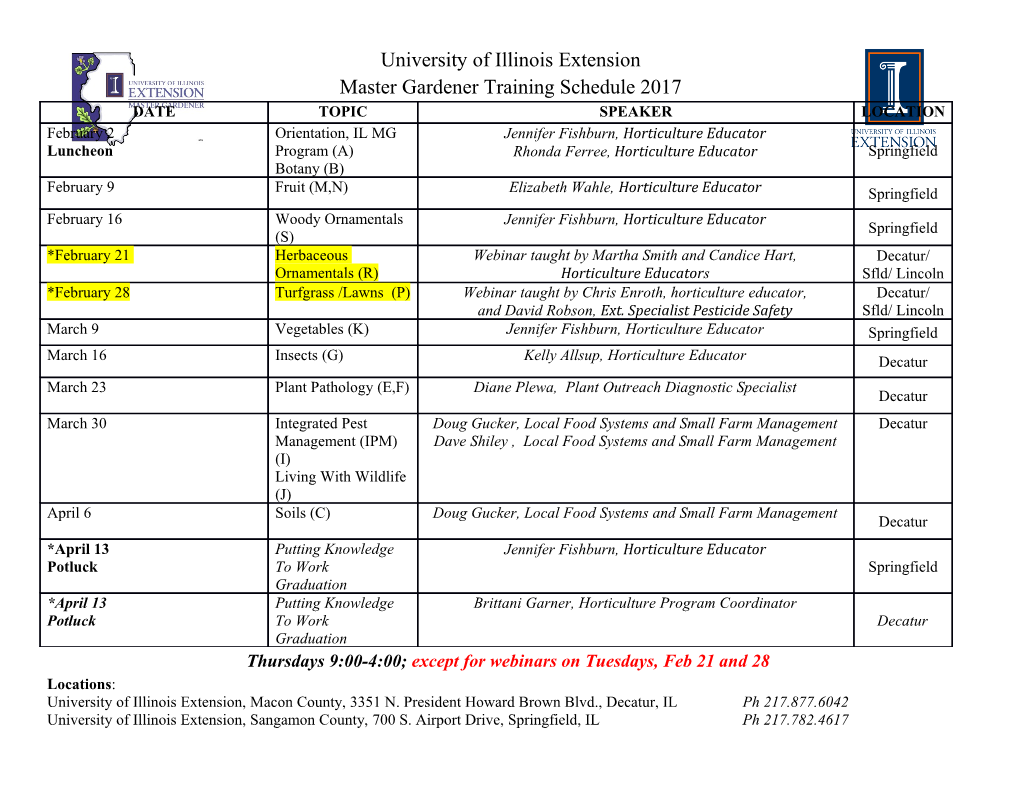
Nanophotonics 1 (2012): 267–291 © 2012 Science Wise Publishing & De Gruyter • Berlin • Boston. DOI 10.1515/nanoph-2012-0021 Review Label-free detection with high-Q microcavities: a review of biosensing mechanisms for integrated devices Frank Vollmer1,* and Lan Yang2 selective detection of biomolecular markers – even against the 1Max Planck Institute for the Science of Light, Laboratory of background of a multitude of other molecular species. Here, it Nanophotonics and Biosensing, G.Scharowsky Str. 1, 91058 is essential to achieve single molecule detection capabi lity in Erlangen, Germany, e-mail: [email protected] an aqueous environment since clinical samples are water based 2Electrical and Systems Engineering Department, and rapid detection relies on transduction of single molecular Washington University, St. Louis, MO 63130, USA interaction events. Although there are many approaches to label-free bio- *Corresponding author sensing only few technologies promise single mole cule Edited by Shaya Fainman, UC San Diego, La Jolla, CA, USA detection capability potentially integrated on a chip-scale platform. Figure 1 shows what we identify as the most Abstract prominent approaches: high-Q optical resonators, plasmon resonance sensors, nanomechanical resonators and nano- Optical microcavities that confi ne light in high-Q resonance wire sensors. In this review we will focus on high-Q optical promise all of the capabilities required for a successful next- resonator-based biosensors and discuss their various mech- generation microsystem biodetection technology. Label-free anisms for biodetection, possibly down to single molecules. detection down to single molecules as well as operation in Similar to the nanomechanical [6, 7], electrical [8, 9] and aqueous environments can be integrated cost-effectively on plasmonic [1] counterparts shown in Figure 1 and Table 1, microchips, together with other photonic components, as well the sensitivity of optical resonators scales inversely with as electronic ones. We provide a comprehensive review of size. In contrast, non-resonant optical detection schemes the sensing mechanisms utilized in this emerging fi eld, their such as those based on Mach-Zehnder interferometers physics, engineering and material science aspects, and their [2, 28] do not. The fabrication of miniature high-Q opti- application to nanoparticle analysis and biomolecular detec- cal microcavities from different materials and in different tion. We survey the most recent developments such as the geometries is therefore a particularly important engineering use of mode splitting for self-referenced measurements, plas- task as we will review in the following. Furthermore, nan- monic nanoantennas for signal enhancements, the use of opti- otechnology-enabled optical microcavities are not only one cal force for nanoparticle manipulation as well as the design of the most sensitive approaches to probing the biological of active devices for ultra-sensitive detection. Furthermore, world in solution, they also are multi-function sensing plat- we provide an outlook on the exciting capabilities of func- forms and we will highlight various mechanisms for sizing, tionalized high-Q microcavities in the life sciences. trapping, and manipulating at the nano-scale [29–32]. 2. Optical resonator-based biomolecular sensors: Keywords: biosensing; integrated photonics; microlasers; optical microcavities; optical trapping; plasmonics; optical mechanisms for detection resonator; nanoparticle detection. 2.1. Light confi nement in high-Q optical microcavities 1. Label-free microsystems biodetection, down For most single-pass optical devices, such as waveguide to single molecules and optical interferometers, light interacts with the analyte molecules or proteins only once. Thus to enhance the inter- Recent years have seen tremendous progress in the deve- action between the light and the sensing target usually one lopment of micro- and nanoscale optical technologies for must increase the physical length of the sensor. However, in biodetection, with research activities mainly focused on devel- a resonator-based sensor where light is confi ned in a micron oping highly sensitive detection schemes. The sensitive and scale sensor element and where the light waves interfere con- label-free detection of biomolecules such as viruses, DNA and structively, we can increase the effective interaction length by proteins, is particularly important for implementing next-gen- increasing the optical quality factor (Q) of the resonant system. eration clinical diagnostic assays. Such assays, implemented The quality factor (Q), which is used to quantify the temporal on chip-scale devices, will replace current labor-intensive and confi nement of the electromagnetic energy is defi ned as expensive laboratory tests. These next generation microchips Ut() Q= are ultimately expected to boast ultimate single molecule ⎛⎞ (1) dU() t ϖ detection capability, will be integrated with other electric and -/⎜⎟0 ⎝⎠dt microfl uidic components, and will be capable of stringent and 268 F. Vollmer and L. Yang: A review of microcavity biosensing mechanisms Optical resonatorPlasmon resonance Mechanical resonator Nanowire Virus Virus Δλ 1 Δλ Virus 2 Figure 1 Prominent microsystem biosensing technologies developed for label-free detection down to single molecules. From left to right: optical resonator, plasmon resonance biosensor, nanomechanical resonator and nanowire sensor. Reproduced with permission from [1–5]. where U(t) is the total energy of the confi ned light fi eld and lifetime of ∼30 ns and can travel in the cavity for ∼10 meters ω -(dU(t)/dt)/ 0 is proportional to the energy that is lost for before it is lost. If the round trip of the photon in the cavity is each electromagnetic oscillation. From this it follows that the 100 μm, the photon will interact with a target molecule more = 5 energy of the charged cavity will decay over time with U(t) U0 than 10 times. This creates a “signal build-up process” inside ω exp(- 0t/Q) once the light source has been shut off. The ring- the resonator that gives rise to signifi cant signal amplifi cation down time τ measured with a photodetector placed in close and allows ultra-sensitive detection of biomolecules. τ = ω proximity to the microcavity, is Q/ 0. The exponentially High-Q optical resonators have been investigated inten- decay of the energy U(t) is characteristic of a resonant system. sively over the past decade as a promising technology for The energy U(t) is proportional to the electromagnetic fi eld ultra-sensitive and label-free biosensing [10, 36]. One of strength squared U(t)∞E(t)2, and it follows that the complex the most promising designs is the whispering-gallery-mode = ω ω| | fi eld evolves in time as: E(t) exp(- 0t/2Q)exp(-i t ). Time (WGM) resonator [37, 38], in which light is trapped in cir- and frequency domains are linked by Fourier transforms: cular orbits by total internal refl ections from the boundar- ∞ ies of the resonator (Figure 2). WGM resonators exhibit 1 ω E()tEed= ∫ ()ωω-it , the highest Q factor [39], up to three orders of magnitude π 2 -∞ higher than other types of resonators. Other types of high-Q WGM-type microcavity biosensors are ring resonators [40], and we therefore expect a spectral response that exhibits a microtoroids [16], and glass capillaries – so called liquid- Lorentzian line: core optical-ring resonators (LCORR) [41]. The ultra-high-Q resonators possess many interesting properties, not available ()ω 2 ∝ 1 in other photonic devices, such as ultra-low loss, ultra-long E 22, ()(ωω-/2+ ω Q ) 00142 43 photon lifetime and ultra-high intracavity power and inten- ==Δωω FWHM0 /Q sity. Due to these unique attributes, the minimum detectable concentration of a target molecule, i.e., the detection limit, ω Δω = ω where 0 is the resonance wavelength and 0/Q is is signifi cantly lower in the ultra-high-Q resonators than in the linewidth (full width at half maximum, FWHM). The other types of sensors. In fact, it has been demonstrated that (total) intrinsic Q-factor of an optical mode is calculated a single virus or nanoparticle can be detected by a WGM ≈ resonator [3, 30]. from 1/Qtotal 1/Qabsorption + 1/Qscattering + 1/Qradiation, where As evidenced in Figure 3, few components are needed 1/Qabsorption denotes losses of photons by material absorption, 1/Qscattering represents scattering losses due to surface inhomo- for an experimental realization of a WGM biosensor. In one geneities or surface defects, and 1/Qradiation is set by the radia- implementation, a continuous-wave tunable distributed feed- tive losses [33]. For optical resonators with negligible amount back laser (DFB), for example operating in the telecom band of losses from radiation such as for example ∼100 μm silica at 1.3 μm wavelength, excites high-Q optical resonances in microsphere, the ultimate material limited Q factor [33, 34] a microsphere via a tapered optical fi ber. The resonances are is close to excited by evanescent coupling from a tapered section of a single mode optical fi ber [43, 44], a method that transfers a 2πn Q =≈glass 1010 , high power fraction from the fi ber to the microcavity. In fact material αλ glass it has been shown that even critical coupling (no transmis- sion at the output of the waveguide on resonance) can be α ∼ The reported optical attenuation coeffi cient of glass 7 achieved if the coupling distance and the taper diameter is db/km (where ∼5 dB is bulk Rayleigh scattering and ∼2 dB carefully controlled [45]. Moreover, tapers can be fabricated is absorption). Low dissipation systems with high Q factors are in automated setups [46]. Once coupled, the microsphere particularly suitable for monitoring perturbations of the reso- spectrum is recorded by sweeping the wavelength of the nance signal [35] and thus for detecting biomolecules [10]. For laser, typically over a narrow spectral range of ∼0.3 nm. It is example, in an optical microsphere-resonator (Figure 2) with a worth noting that the Q factor is now further modifi ed by the Q-factor of 108 a photon (with a wavelength of 600 nm) has a coupling Q factor [47].
Details
-
File Typepdf
-
Upload Time-
-
Content LanguagesEnglish
-
Upload UserAnonymous/Not logged-in
-
File Pages25 Page
-
File Size-