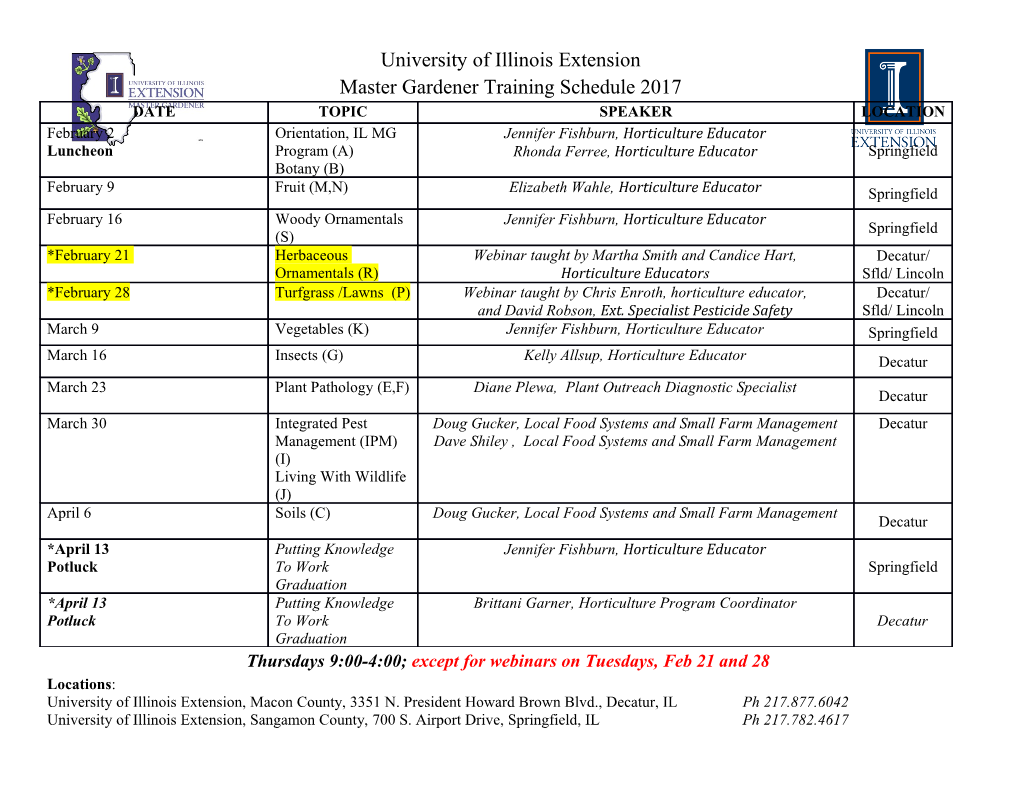
<p> 1 Strengthening mechanisms in nanostructured Al/SiCp composite</p><p>2 manufactured by accumulative press bonding</p><p>3 4 Sajjad Amirkhanlou a,b,c,*, Mehdi Rahimian c,d, Mostafa Ketabchi a, Nader Parvin a, Parisa</p><p>5 Yaghinali a, Fernando Carreño b</p><p>6 7 a Department of Mining and Metallurgical Engineering, Amirkabir University of Technology, Tehran, </p><p>8 Iran</p><p>9 b Department of Physical Metallurgy, CENIM-CSIC, Av. Gregorio del Amo 8, 28040 Madrid, Spain</p><p>10 c Institute of Materials and Manufacturing, Brunel University London, London UB8 3PH, United </p><p>11 Kingdom </p><p>12 d IMDEA Materials Institute, C/Eric Kandel 2, 28906, Getafe, Madrid, Spain</p><p>13 14 * Corresponding author: Email: [email protected]; [email protected] 15</p><p>16</p><p>17 Abstract</p><p>18 The strengthening mechanisms in nanostructured Al/SiCp composite deformed to </p><p>19 high strain by a novel severe plastic deformation process, accumulative press </p><p>20 bonding (APB), was investigated. The composite exhibited yield strength of 148 </p><p>21 MPa which was 5 and 1.5 times higher than that of raw aluminum (29 MPa) and </p><p>22 aluminum-APB (95 MPa) alloys, respectively. A remarkable increase was also </p><p>23 observed in the ultimate tensile strength of Al/SiCp-APB composite, 222 MPa, </p><p>24 which was 2.5 and 1.2 times greater than the obtained values for raw aluminum </p><p>25 (88 MPa) and aluminum-APB (180 MPa) alloys, respectively. Analytical models </p><p>26 well described the contribution of various strengthening mechanisms. The </p><p>27 contribution of grain boundary, strain hardening, thermal mismatch, Orowan, </p><p>28 elastic mismatch and load-bearing strengthening mechanisms to the overall </p><p>29 strength of the Al/SiCp micro-composite were 64.9, 49, 6.8, 2.4, 5.4 and 1.5 MPa, </p><p>30 respectively. Whereas Orowan strengthening mechanism was considered as the 2</p><p>31 most dominating strengthening mechanism in Al/SiCp nanocomposites, it was </p><p>32 negligible for strengthening of the micro-composite. Al/SiCp nanocomposite </p><p>33 showed good agreement with quadratic summation model; however, experimental </p><p>34 results exhibited a good accordance with arithmetic and compounding summation </p><p>35 models in the micro-composite. While average grain size of the composite reached</p><p>36 380 nm, it was less than 100 nm in the vicinity of SiC particles as a result of </p><p>37 particle stimulated nucleation mechanism. </p><p>38 39 Keywords: Accumulative press bonding (APB); Severe plastic deformation (SPD);</p><p>40 Strengthening mechanisms; Analytical models; Metal matrix composites; </p><p>41 Nanostructured materials</p><p>42</p><p>43 1. Introduction</p><p>44 Aluminum matrix composites (AMCs), reinforced with particulate reinforcement, </p><p>45 have attracted considerable attention in automotive and aerospace industries, due </p><p>46 to their low weight and high mechanical properties [1, 2]. Silicon carbide (SiCp) is </p><p>47 considered as a typical cost effective particulate reinforcement used widely in </p><p>48 AMCs because of its high strength and modulus [3, 4]. Traditional processing </p><p>49 routes for fabrication of Al/SiCp composite, including casting, powder metallurgy </p><p>50 and spray forming encounter various shortcomings. The main drawbacks of those </p><p>51 liquid state techniques [5, 6] can be referred as SiCp agglomeration, weak </p><p>52 adhesion and undesirable chemical reaction occurred between Al and SiCp [7, 8]. </p><p>53 However, manufacturing techniques in solid state can overcome the above </p><p>54 problems [9-11]. Microstructure and mechanical properties of Al/SiCp composite, </p><p>55 manufactured by accumulative roll bonding (ARB) as a solid-state process, was 3</p><p>56 evaluated by Jamaati et al. [12-14]. Accumulative press bonding (APB), introduced </p><p>57 for the first time in our previous works, is another severe plastic deformation </p><p>58 process [15, 16] enabling us to fabricate particle reinforced AMCs. Uniform </p><p>59 distribution of reinforcement, nano/ultra-fine structure and high mechanical </p><p>60 properties are obtained using APB process [17-20]. Many researches were focused</p><p>61 on the fabrication and characterization Al/SiCp composites prepared by metal </p><p>62 forming processes [21, 22]. However, individual contributions of various </p><p>63 micromechanics strengthening factors in AMCs deformed to high strain were not </p><p>64 investigated in previous studies. In this study the novel APB process was utilized </p><p>65 for fabrication of Al/SiCp composite and the effect and proportion of various </p><p>66 strengthening mechanisms on the final yield strength was assessed. Moreover, </p><p>67 advanced microstructural characterization techniques were employed to verify </p><p>68 each strengthening mechanism.</p><p>69</p><p>70 2. Experimental procedure</p><p>71 As-received AA1050 aluminum sheets, chemical composition is given in Table 1, </p><p>72 and SiC particles with an average size of 10 m were used as raw materials. </p><p>73 Aluminum sheets with the dimensions of 100 mm 50 mm 1.5 mm were </p><p>74 annealed at 623 K (350 ºC) for 1 h. The accumulative press bonding (APB) </p><p>75 process for manufacturing of the Al/10 vol.% SiCp composite was schematically </p><p>76 reported in ref. [23, 24]. The aluminum sheets were degreased in acetone bath </p><p>77 followed by scratch brushing with 0.4 mm wire diameter and peripheral speed of </p><p>78 2800 rpm. The reinforcement particles were uniformly spread between surfaces by</p><p>79 a hand sprayer. A hydraulic press machine was utilized to form a mechanical bond</p><p>80 between two stacked sheets, in a channel die, where the thickness of sheets 4</p><p>81 reduced by 50%. The APB process was performed at ambient temperature. The </p><p>82 fabricated sheet was cut in two pieces and the whole mentioned process was </p><p>83 repeated 5 times in order to increase SiC particles to 10 vol.%. Thereafter, the </p><p>84 above process was repeated 7 times but without any reinforcement addition. The </p><p>85 same process was employed for the production of the monolithic aluminum in </p><p>86 which the aluminum sheets were processed by APB without adding any SiCp </p><p>87 powder through the process.</p><p>88 Tensile tests were performed according to ASTM E8 standard at a rate of 1.6 by </p><p>89 a Houndsfield H50KS machine. The gauge width, thickness and length of </p><p>90 specimens were 6, 1.5 and 25 mm, respectively. Various microstructural aspects </p><p>91 of specimens were investigated by transmission electron microscopy (TEM, JEOL </p><p>92 JEM 2000 FX II, JEOL Ltd. Tokyo, Japan) operating at 200 kV and field-emission </p><p>93 scanning transmission electron microscopy (FE-STEM, HITACHI S-4800, Hitachi </p><p>94 Ltd., Tokyo, Japan) operating at 10 kV complemented by energy-dispersive </p><p>95 spectroscopy (EDS, 10mm2 SDD Detector X-ACT, Oxford instrument, Oxford, </p><p>96 England). Also the grain boundary characterization was performed by electron </p><p>97 backscattered diffraction (EBSD, JEOL JSM 6500 F) adjusted at 20 kV with a </p><p>98 working distance of 15 mm, step size of 80 nm and tilt angle of 70º. Thin foils </p><p>99 required for EBSD, TEM and STEM investigations were mechanically ground and </p><p>100 punched into 3 mm discs with an average thickness of less than 100 μm. The discs </p><p>101 were subsequently thinned to perforation using a twin-jet electropolishing facility </p><p>102 (TenuPol-5, Struers) with a solution of 30% nitric acid and 70% methanol at 11 V </p><p>103 and 245 K (−28 ºC). The X-ray pattern of the manufactured Al/SiCp composite was </p><p>104 recorded with an X-ray diffractometer (XRD). The XRD experiment was conducted by a </p><p>105 Philips X’PERT MPD X-ray diffractometer with CuKα radiation in the range of using a 5</p><p>106 step size of and a counting time of 1 s per step. Consequently, XRD patterns were </p><p>107 analyzed via X’Pert HighScore software.</p><p>108</p><p>109 3. Results and discussion</p><p>110 The stress-strain curves of annealed aluminum (Al), monolithic aluminum (Al-APB)</p><p>111 and Al/SiCp-APB composite are shown in Figure 1. According to the Figure 1, the </p><p>112 yield strength of the aluminum, which is 29 MPa, was improved by 5 times, as it </p><p>113 increases to 148 MPa. A remarkable increase was also observed in the ultimate </p><p>114 tensile strength of Al/SiCp-APB composite, 222 MPa, which was 2.5 and 1.2 times </p><p>115 greater than the obtained values for raw aluminum (88 MPa) and aluminum-APB </p><p>116 (180 MPa) alloys, respectively. Although this study has not been done previously, </p><p>117 relevant composites fabricated via other production processes are summarized </p><p>118 in Table 2. The superior strength of the produced composite through APB process </p><p>119 is obtained mainly due to the uniform distribution of particles, formation of ultra-fine</p><p>120 structure and low level of porosity. The enhancement of composite’s strength can </p><p>121 be described by different mechanisms. In following sections, microstructural </p><p>122 evidences and theoretical models are employed to explain each strengthening </p><p>123 mechanism.</p><p>124</p><p>125 3.1- Grain boundary </p><p>126 Figure 2 shows STEM micrographs of Al/SiCp composite after various cycles of </p><p>127 APB process. It is observed that gradual grain refining occurred during process </p><p>128 and grains are slightly elongated in the longitudinal direction. Average grain size </p><p>129 reduced to 380 nm after 14 cycles of APB, Figure 2e. Grain refining is the most </p><p>130 desirable strengthening mechanism because it is only mechanism which leads to 6</p><p>131 simultaneous increment of strength and toughness [25, 26]. The formation </p><p>132 mechanism of nano grains by the APB process is considered as continuous </p><p>133 dynamic recovery (CDR). In CDR the size of small (sub) grains remains constant, </p><p>134 whereas grains misorientation increases. In fact, there isn’t any nucleation and </p><p>135 growth of deformed nuclei in CDR, because the dislocations glide directly from one</p><p>136 side of grain to the other side resulting in the increment of grains misorientation. </p><p>137 This is the most equilibrated way of obtaining the finest and sharpest histogram of </p><p>138 grain sizes, which leads to the highest misorientation for the given processing </p><p>139 conditions. The grain refinement mechanisms of pure aluminum under APB </p><p>140 process were discussed in our previous studies [19, 20]. However, two other </p><p>141 factors encourage CDR of Al/SiCp composite including severe shear deformation </p><p>142 and micro-size particles. In fact, finer grain size can be obtained in APB process </p><p>143 on account of the present of non-deformable reinforcements. Figure 3 displays the</p><p>144 interface of the SiC particle and aluminum matrix. The finer grain sizes are </p><p>145 recognized in the vicinity of SiC particles where the average grain size measured </p><p>146 less than 100 nm. When the composite is exposed by deformation during the </p><p>147 process, the existence of non-deformable particles induces strain to their vicinity. </p><p>148 As a result, the vicinity of particles is fertilized to form new boundaries due to the </p><p>149 introduction of a high dislocation density, referred as particle stimulated nucleation </p><p>150 (PSN) [27, 28]. The accumulation of dislocations in the vicinity of particles </p><p>151 facilitated the formation of fine grains by continuous dynamic recovery mechanism.</p><p>152 Consequently, the average grain size of the composite, 380 nm, is finer than that </p><p>153 of monolithic aluminum which is 450 nm [19]. Other factor, considered for grain </p><p>154 refinement of pure aluminum and the composite, is severe shear deformation. </p><p>155 TEM micrographs of surface and center of the monolithic aluminum after one APB 7</p><p>156 cycle are shown in Figure 4. Comparison of Figure 4a and b demonstrates the </p><p>157 higher density of dislocation tangle zones on the surface. This observation is </p><p>158 attributed to the severe shear strain exists between the sample and press anvil. In </p><p>159 each APB cycle, the surface containing higher dislocation density is moved toward</p><p>160 the center resulting in homogeneous distribution of dislocation through the bulk </p><p>161 material. Therefore, dislocations formed because of severe shear contribute to the </p><p>162 final grain refinement. Grain boundary strengthening () can be explained by well-</p><p>163 known Hall-Petch equation (Eq. 1) [29]. Higher fractions of grain boundaries </p><p>164 existing in finer grain structures increase the number of obstacles against </p><p>165 dislocation movement. </p><p>166 (1)</p><p>167 where is average grain size, is constant and typically equal to 40 MPa for </p><p>168 aluminum alloys [19, 30]. While the grain boundary strengthening was calculated </p><p>169 5.2 MPa for Al [20], it increased to 59.6 MPa and 64.9 , for Al-APB and Al/SiCp </p><p>170 composite, respectively. </p><p>171</p><p>172 3.2- Thermal mismatch (TM) </p><p>173 Discrepancy of thermal expansion coefficient (CTE) between matrix and </p><p>174 reinforcement acts as a dislocation generation source [31, 32]. Since, thermal </p><p>175 expansion coefficient of the matrix, , differs from the SiCp reinforcement, , strain is </p><p>176 induced to the matrix around the particles resulting in dislocation formation, as </p><p>177 shown in Figure 5a. Multi-directional thermal stresses at the particle/matrix </p><p>178 interface, which are induced by the difference of thermal expansion between </p><p>179 aluminum and SiC particles, result in mismatch strain around the particles. The </p><p>180 system makes an attempt to reduce internal energy, mismatch strain, via 8</p><p>181 introducing new dislocations [33, 34]. High dislocation density in the vicinity of </p><p>182 particles, observed in Figure 5a, can arrange and form new grain boundaries via </p><p>183 continuous dynamic recovery during APB process, as shown in Figure 5b. </p><p>184 Strengthening effect of thermal mismatch () can be expressed by the following </p><p>185 equations [35, 36]:</p><p>186 (2)</p><p>187 where is shear modulus (~25.4 GPa for aluminum) and is the average value of </p><p>188 dislocation strengthening efficiency (∼1 for pure metals [37]) and is the Burgers </p><p>189 vector (=0.286 nm [38]). Dislocation density, resulted from CTE mismatch, is </p><p>190 governed by particles volume fraction, , difference between processing and </p><p>191 ambient temperature, [39], and variation between CTE of particles and matrix, . </p><p>192 Dislocation density induced by thermal mismatch can be calculated by [40]: </p><p>193 (3)</p><p>194 The amount of is calculated around 6.8 MPa for Al/SiCp composite, while this </p><p>195 mechanism is not taken into account for Al and Al-APB alloys.</p><p>196</p><p>197 3.3- Elastic mismatch (EM)</p><p>198 The difference of elastic modulus between matrix and reinforcement introduce an </p><p>199 additional dislocation into the composite in order to reduce induced plastic strain. </p><p>200 The density of generated dislocation due to elastic modulus mismatch can be 9</p><p>201 estimated by Eq. (4). These dislocations induce additional strength to the </p><p>202 composite which is expressed by Eq. (5) [41]:</p><p>203 (4)</p><p>204 (5)</p><p>205 where is yield strain (0.2%) and is density of dislocations caused by elastic </p><p>206 mismatch [42]. Whereas, due to absence of reinforcement in Al and Al-APB, there </p><p>207 is no elastic mismatch strengthening effect, it is calculated around 5.4 for Al/SiCp </p><p>208 composite. </p><p>209</p><p>210 3.4- Strain hardening</p><p>211 Figure 6 displays EBSD/orientation imaging microscopy (OIM) and grain boundary</p><p>212 maps of Al/SiCp composite. The red/gray lines correspond to the low angle grain </p><p>213 boundaries (LAGBs) having misorientations 2-15º, and the high angle grain </p><p>214 boundaries (HAGBs) are shown as black lines which have misorientations above </p><p>215 15º. The fraction of high angle grain boundaries () and the mean misorientation </p><p>216 angle of the boundaries () for the Al/SiCp composite were 73% and 35º, </p><p>217 respectively. According to EBSD results, it is obvious that APB process had a </p><p>218 significant effect on the development of an ultra-fine grain structure surrounded </p><p>219 mainly by high-angle boundaries. Formation of the well-developed high angle </p><p>220 boundary during APB process is attributed to the rearrangement of the </p><p>221 dislocations via short-range diffusion [43-46]. As a result of mechanical </p><p>222 deformation, dislocations will be generated resulting in the increment of strength. It</p><p>223 is well known that dislocations tend to array and form low angle grain boundaries </p><p>224 during severe plastic deformation process. Therefore, low angle grain boundaries </p><p>225 can be considered as a dislocation resource. In other word, HAGBs contribute to 10</p><p>226 the grain boundary strengthening mechanism which is determined by Hall-Petch </p><p>227 relation, whereas dislocation strengthening mechanism is related to LAGBs, as </p><p>228 explained by Hansen et al. [47]. The strength imposed by LAGBs to the system is </p><p>229 expressed by:</p><p>230 (6)</p><p>231 where is the dislocation strengthening efficiency (the average value = 0.24) and </p><p>232 M is the Taylor factor (for aluminum is 3.06). Following equation shows the density</p><p>233 of dislocations introduced by LAGBs to the system [48, 49]:</p><p>234 (7)</p><p>235 where , and are the mean misorientation of LAGBs, volume fraction of HAGBs and</p><p>236 average LAGBs spacing that is measured from EBSD results. is 8, 47 and 49 </p><p>237 MPa for initial aluminum, Al-APB and Al/SiCp composite processed by APB, </p><p>238 respectively.</p><p>239</p><p>240 3.5- Orowan strengthening</p><p>241 Orowan mechanism corresponds to the interaction of the particles and dislocations</p><p>242 in which nano particles pin dislocations resulting in bowing dislocation around </p><p>243 particles and create Orowan rings. Increment of yield strength, in polycrystalline </p><p>244 materials, induced by Orowan mechanisms can be calculated by [41, 50]: </p><p>245 (8)</p><p>246 where is the Poisson’s ratio (0.33). A small contribution of Orowan strengthening </p><p>247 mechanism, , in Al/SiCp micro-composite can be interpreted by large distance of </p><p>248 micro-size particles.</p><p>249</p><p>250 3.6- Load-bearing 11</p><p>251 FE-SEM micrographs of Al/SiCp composite after several APB cycles are shown in </p><p>252 then Figure 7. With increasing number of cycles, the laminar structure is converted</p><p>253 into the homogeneous structure. The formation mechanism of this structure is </p><p>254 explained comprehensively in our previous study [17, 18]. It should be briefly </p><p>255 pointed out that aluminum plastic flow, because of applied stress during APB, led </p><p>256 to refinement and dispersion of SiCp clusters. The high pressures associated with </p><p>257 APB resulted in the squeezing of the Al-matrices within the SiCp clusters producing</p><p>258 homogenous structure. Formation of strong bond between the particles and matrix</p><p>259 due to extensive pressure can be another advantage of current process. Since, in </p><p>260 the tensile test a fraction of stress is transferred to particles, having higher </p><p>261 modulus and strength compared with matrix, composite can withstand higher load </p><p>262 than monolithic aluminum. In order to achieve maximum potential of load-bearing </p><p>263 effect, homogeneous distributed particles having strong bond with matrix are </p><p>264 required. Figure 8 displays SEM micrographs of Al/SiCp composite produced by </p><p>265 APB together with its EDS and X-ray maps. Al4C3 phase, observed usually in the </p><p>266 cast Al/SiCp composites, exhibits detrimental effect on interfacial bonding and </p><p>267 mechanical properties on account of its brittle nature [51]. The X-ray maps (Figure </p><p>268 8b-f) and X-ray diffraction pattern (Figure 9) show that there is no evidence of </p><p>269 undesired phase such as Al4C3 in the microstructure considered as the advantage </p><p>270 of solid state fabrication of Al/SiCp composite by the current process.</p><p>271 Well distributed particles endure a proportion of applied force imposed directly by </p><p>272 tensile test. The contribution of load-bearing mechanism in increasing of yield </p><p>273 strength is expressed by Eq. 9, which is the modification of shear-lag model:</p><p>274 (9) 12</p><p>275 where and are referred to volume fraction of particles and matrix yield strength, </p><p>276 respectively. is 1.5 MPa for Al/SiCp composite. </p><p>277 The total yield strength is calculated by three well-known models referred as </p><p>278 arithmetic summation (Eq. 10), quadratic summation (Eq. 11) and compounding </p><p>279 methods (Eq. 12) [41, 52, 53]:</p><p>280 (10)</p><p>281 (11)</p><p>282 (12)</p><p>283 Contribution of the various strengthening mechanisms as well as yield strength, </p><p>284 obtained by various models and tensile tests, are displayed in Table 3. The </p><p>285 influence of each factor on yield strength of micro-composite is evaluated against </p><p>286 that of nanocomposite, which was investigated in our previous study [20].</p><p>287 Matrix flow through micro-particles is easier than nanoparticles so nanocomposite </p><p>288 is associated with smaller grain (280 nm) compare with composites reinforced with</p><p>289 micro-particles (380 nm). Therefore, improvement of yield strength due to grain </p><p>290 boundary mechanism is 75.6 MPa for nanocomposite, while this value is 64.9 for </p><p>291 Al/SiCp micro-composite. Although grain boundaries strengthening mechanism has</p><p>292 conquered the second place enhancing mechanical properties in the </p><p>293 nanocomposite, it is promoted to first place in the micro-size composite. By </p><p>294 decreasing volume fraction of reinforcement/matrix interfaces in the macro-</p><p>295 composite compare with the nanocomposite, dislocation density formed in the </p><p>296 matrix of micro-composite due to thermal and elastic mismatch is significantly </p><p>297 decreased. Whereas Orowan strengthening mechanism was considered as the </p><p>298 most important strengthening mechanism in nanocomposites, it is negligible for </p><p>299 strengthening of the micro-size composites. As a result of large size and distance 13</p><p>300 of reinforcement, grains and subgrains interact with dislocations instead of </p><p>301 interacting with SiC particles. Strain hardening and grain boundary strengthening </p><p>302 mechanisms are considered as the two most effective strengthening mechanisms </p><p>303 in Al/SiCp micro-composite. The load transfer effect in both composites is </p><p>304 negligible because of particulate shape and low volume fraction of reinforcement. </p><p>305 Since the number of active strengthening mechanisms in Al/SiCp nanocomposite is</p><p>306 considerably higher than the micro-composite, the final experimental yield strength</p><p>307 of the nanocomposite increased up to 210 MPa. Based on the result of </p><p>308 calculations performed by each model, it is understood that experimental result </p><p>309 exhibits a good accordance with arithmetic summation and compounding models </p><p>310 in micro-composite. However, nanocomposite shows good agreement with </p><p>311 quadratic summation model, as demonstrated in previous study [20]. Short </p><p>312 dislocation gliding distance in the nanocomposites imposed by well distributed </p><p>313 nanoparticles and concomitant very fine grains results in the overestimating of </p><p>314 calculated results compared with experimental one. In other words, the first </p><p>315 obstacle on the way of dislocation movement, which can be LAGBs, HAGBs or </p><p>316 nanoparticles, leads to the strengthening of nanocomposite. Therefore, it is </p><p>317 expected that considering the contribution of strain hardening (LAGBs), grain </p><p>318 boundaries (HAGBs) and Orowan (nanoparticles) mechanisms together in </p><p>319 strengthening of nanocomposite, exhibiting an overestimation of the resistance of </p><p>320 the alloy.</p><p>321</p><p>322 4. Conclusions</p><p>323 In the present investigation, the micromechanics strengthening in nanostructured </p><p>324 Al/SiCp composite deformed to high strain by a novel severe plastic deformation 14</p><p>325 process, accumulative press bonding (ARB), was investigated. The improvement </p><p>326 in yield strength of Al/SiCp composite was described by various strengthening </p><p>327 mechanisms. Advanced microstructural techniques were employed to present </p><p>328 evidences of each strengthening mechanism. The conclusions drawn from the </p><p>329 results can be summarized as follows:</p><p>330 1) Homogeneous distribution of SiC particles (with average particle size of 10 </p><p>331 µm) was successfully achieved after 14 cycles of APB process.</p><p>332 2) The EDS maps and X-ray diffraction pattern showed that there was no </p><p>333 evidence of detrimental phases in the microstructure of Al/SiCp composite </p><p>334 considered as the advantage of solid state fabrication process. </p><p>335 3) Nanostructured Al/SiCp composite with the average grain size of 380 nm and </p><p>336 well-developed high-angle grain boundaries (73% high angle boundaries and </p><p>337 35° average misorientation angle) was obtained by performing 14 cycles of </p><p>338 APB process.</p><p>339 4) As a result of particle stimulated nucleation mechanism, grain size of the </p><p>340 composite was less than 100 nm in the vicinity of SiC particles.</p><p>341 5) The yield strength of the aluminum, being 29 MPa, was improved by 5 times, </p><p>342 as it increased to 148 MPa. </p><p>343 6) The contribution of grain boundary, strain hardening, thermal mismatch, </p><p>344 Orowan, elastic mismatch and load-bearing strengthening mechanisms were </p><p>345 64.9, 49, 6.8, 2.4, 5.4 and 1.5 MPa, respectively. Clearly, strain hardening and </p><p>346 grain boundary mechanisms demonstrate higher contribution to the overall </p><p>347 strength of the Al/SiCp composite.</p><p>348 7) Al/SiCp nanocomposite showed good agreement with quadratic summation </p><p>349 model, however, based on the result of calculations performed by each model, </p><p>350 it is understood that experimental result exhibits a good accordance with </p><p>351 arithmetic and compounding summation models in micro-composite. 15</p><p>352</p><p>353 Acknowledgment</p><p>354 The authors acknowledge financial support from CICYT (Spain) under program </p><p>355 MAT2012-38962-C03-01, and the Ministry of Science, Research and Technology </p><p>356 of Iran.</p><p>357 </p><p>358 16</p><p>359 References</p><p>360 [1] R. Jamaati, M. Naseri, M.R. Toroghinejad: Mater. Des., 2014, vol. 59, pp. 540-549.</p><p>361 [2] R. Jamaati, M.R. Toroghinejad, J. Dutkiewicz, J.A. Szpunar: Mater. Des., 2012, vol. </p><p>362 35, pp. 37-42.</p><p>363 [3] I. Ibrahim, F. Mohamed, E. Lavernia: J. Mater. Sci., 1991, vol. 26, pp. 1137-1156.</p><p>364 [4] E. Lacoste, C. Arvieu, J.M. Quenisset: J. Mater. Sci., 2015, vol. 50, pp. 5583-5592.</p><p>365 [5] H. Yue, H. Zhang, X. Gao, J. Chang, S. Zhang, J. Zhang, E. Guo, L. Wang, Z. Yu: </p><p>366 Mater. Charact., 2015, vol. 99, pp. 47-51.</p><p>367 [6] Š. Nagy, M. Nosko, Ľ. Orovčík, K. Iždinský, S. Kúdela, P. Krížik: Mater. Des., 2015, </p><p>368 vol. 66, pp. 1-6.</p><p>369 [7] S. Amirkhanlou, B. Niroumand: J. Mater. Process. Technol., 2012, vol. 212, pp. 841-</p><p>370 847.</p><p>371 [8] S. Amirkhanlou, B. Niroumand: J. Mater. Eng. Perform., 2013, vol. 22, pp. 85-93.</p><p>372 [9] Y. Ikoma, T. Toyota, Y. Ejiri, K. Saito, Q. Guo, Z. Horita: J. Mater. Sci., 2015, vol. 51,</p><p>373 pp. 138-143.</p><p>374 [10] M. Kawasaki, T.G. Langdon: J. Mater. Sci., 2015, vol. 51, pp. 19-32.</p><p>375 [11] S. Sabbaghianrad, T.G. Langdon: J. Mater. Sci., 2015, vol. 50, pp. 4357-4365.</p><p>376 [12] R. Jamaati, S. Amirkhanlou, M.R. Toroghinejad, B. Niroumand: Mater. Sci. Eng. A, </p><p>377 2011, vol. 528, pp. 2143-2148.</p><p>378 [13] S. Amirkhanlou, R. Jamaati, B. Niroumand, M.R. Toroghinejad: Mater. Sci. Eng. A, </p><p>379 2011, vol. 528, pp. 4462-4467.</p><p>380 [14] R. Jamaati, S. Amirkhanlou, M.R. Toroghinejad, B. Niroumand: J. Mater. Eng. </p><p>381 Perform., 2012, vol. 21, pp. 1249-1253.</p><p>382 [15] M.I. Latypov, M.G. Lee, Y.E. Beygelzimer, D. Prilepo, Y. Gusar, H.S. Kim: Metall. </p><p>383 Mater. Trans. A, 2016, vol. 47, pp. 1248-1260. 17</p><p>384 [16] Y. Zhang, S. Sabbaghianrad, H. Yang, T.D. Topping, T.G. Langdon, E.J. Lavernia, </p><p>385 J.M. Schoenung, S.R. Nutt: Metall. Mater. Trans. A, 2015, vol. 46, pp. 5877-5886.</p><p>386 [17] S. Amirkhanlou, M. Ketabchi, N. Parvin, S. Khorsand, R. Bahrami: Mater. Des., </p><p>387 2013, vol. 51, pp. 367-374.</p><p>388 [18] S. Amirkhanlou, M. Ketabchi, N. Parvin, G. Drummen: Metall. Mater. Trans. B, </p><p>389 2014, vol. 45, pp. 1992-1999.</p><p>390 [19] S. Amirkhanlou, M. Askarian, M. Ketabchi, N. Azimi, N. Parvin, F. Carreño: Mater. </p><p>391 Charact., 2015, vol. 109, pp. 57-65.</p><p>392 [20] S. Amirkhanlou, M. Ketabchi, N. Parvin, A. Orozco-Caballero, F. Carreño: Scripta </p><p>393 Mater., 2015, vol. 100, pp. 40-43.</p><p>394 [21] M. Rezayat, A. Akbarzadeh, A. Owhadi: Metall. Mater. Trans. A, 2012, vol. 43, pp. </p><p>395 2085-2093.</p><p>396 [22] A. Ahmadi, M.R. Toroghinejad, A. Najafizadeh: Mater. Des., 2014, vol. 53, pp. 13-</p><p>397 19.</p><p>398 [23] S. Amirkhanlou, M. Ketabchi, N. Parvin, M. Askarian, F. Carreño: Mater. Sci. Eng. </p><p>399 A, 2015, vol. 627, pp. 374-380.</p><p>400 [24] S. Amirkhanlou, M. Ketabchi, N. Parvin, S. Khorsand, R. Bahrami: Mater. Des., </p><p>401 2013, vol. 51, pp. 367-374.</p><p>402 [25] A. Mishra, B. Kad, F. Gregori, M. Meyers: Acta Mater., 2007, vol. 55, pp. 13-28.</p><p>403 [26] R. Jamaati, M.R. Toroghinejad, S. Amirkhanlou, H. Edris: Metall. Mater. Trans. A, </p><p>404 2015, vol. 46, pp. 4013-4019.</p><p>405 [27] J. Robson, D. Henry, B. Davis: Acta Mater., 2009, vol. 57, pp. 2739-2747.</p><p>406 [28] Y. Shen, R. Guan, Z. Zhao, R. Misra: Acta Mater., 2015, vol. 100, pp. 247-255.</p><p>407 [29] R. Jamaati, M.R. Toroghinejad, S. Amirkhanlou, H. Edris: Mater. Sci. Eng. A, 2015, </p><p>408 vol. 639, pp. 656-662. 18</p><p>409 [30] M. Alizadeh: J. Alloys Compd., 2011, vol. 509, pp. 2243-2247.</p><p>410 [31] J.G. Park, D.H. Keum, Y.H. Lee: Carbon, 2015, vol. 95, pp. 690-698.</p><p>411 [32] S. Dong, J. Zhou, D. Hui, Y. Wang, S. Zhang: Composites Part A: Applied Science </p><p>412 and Manufacturing, 2015, vol. 68, pp. 356-364.</p><p>413 [33] D. Cheng, J. Niu, Z. Gao, P. Wang: Mod. Phys. Lett. B, 2015, vol. 29, pp. 1540002.</p><p>414 [34] Z. Ni, H. Zhao, F. Ye: Vacuum, 2015, vol. 120, pp. 101-106.</p><p>415 [35] W. Kim, I. Park, S. Han: Scripta Mater., 2012, vol. 66, pp. 590-593.</p><p>416 [36] F. Chen, Z. Chen, F. Mao, T. Wang, Z. Cao: Mater. Sci. Eng. A, 2015, vol. 625, pp. </p><p>417 357-368.</p><p>418 [37] W. Miller, F. Humphreys: Scripta metallurgica et materialia, 1991, vol. 25, pp. 33-38.</p><p>419 [38] K. Edalati, D. Akama, A. Nishio, S. Lee, Y. Yonenaga, J.M. Cubero-Sesin, Z. Horita: </p><p>420 Acta Mater., 2014, vol. 69, pp. 68-77.</p><p>421 [39] S. Lee, Y. Saito, T. Sakai, H. Utsunomiya: Mater. Sci. Eng. A, 2002, vol. 325, pp. </p><p>422 228-235.</p><p>423 [40] R. Arsenault, N. Shi: Mater. Sci. Eng., 1986, vol. 81, pp. 175-187.</p><p>424 [41] L. Jiang, H. Yang, J.K. Yee, X. Mo, T. Topping, E.J. Lavernia, J.M. Schoenung: Acta </p><p>425 Mater., 2016, vol. 103, pp. 128-140.</p><p>426 [42] L. Dai, Z. Ling, Y. Bai: Compos. Sci. Technol., 2001, vol. 61, pp. 1057-1063.</p><p>427 [43] Y. Saito, N. Tsuji, H. Utsunomiya, T. Sakai, R.G. Hong: Scripta Mater., 1998, vol. 39,</p><p>428 pp. 1221-1227.</p><p>429 [44] S.H. Lee, Y. Saito, N. Tsuji, H. Utsunomiya, T. Sakai: Scripta Mater., 2002, vol. 46, </p><p>430 pp. 281-285.</p><p>431 [45] N. Tsuji, Y. Ito, Y. Saito, Y. Minamino: Scripta Mater., 2002, vol. 47, pp. 893-899.</p><p>432 [46] T. Yu, N. Hansen, X. Huang: Acta Mater., 2013, vol. 61, pp. 6577-6586.</p><p>433 [47] N. Hansen: Scripta Mater., 2004, vol. 51, pp. 801-806. 19</p><p>434 [48] J.R. Bowen, P. Prangnell, D.J. Jensen, N. Hansen: Mater. Sci. Eng. A, 2004, vol. 387, </p><p>435 pp. 235-239.</p><p>436 [49] N. Kamikawa, X. Huang, N. Tsuji, N. Hansen: Acta Mater., 2009, vol. 57, pp. 4198-</p><p>437 4208.</p><p>438 [50] Z. Zhang, D. Chen: Mater. Sci. Eng. A, 2008, vol. 483, pp. 148-152.</p><p>439 [51] J.-C. Lee, J.-Y. Byun, S.-B. Park, H.-I. Lee: Acta Mater., 1998, vol. 46, pp. 1771-</p><p>440 1780.</p><p>441 [52] Z. Zhang, D. Chen: Scripta Mater., 2006, vol. 54, pp. 1321-1326.</p><p>442 [53] C.-S. Kim, I. Sohn, M. Nezafati, J. Ferguson, B.F. Schultz, Z. Bajestani-Gohari, P.K. </p><p>443 Rohatgi, K. Cho: J. Mater. Sci., 2013, vol. 48, pp. 4191-4204.</p><p>444 [54] S.A. Sajjadi, H. Ezatpour, M.T. Parizi: Mater. Des., 2012, vol. 34, pp. 106-111.</p><p>445 [55] S. Amirkhanlou, B. Niroumand: Mater. Des., 2011, vol. 32, pp. 1895-1902.</p><p>446 [56] C. Tekmen, I. Ozdemir, U. Cocen, K. Onel: Mater. Sci. Eng. A, 2003, vol. 360, pp. </p><p>447 365-371.</p><p>448 [57] F. Khodabakhshi, H.G. Yazdabadi, A. Kokabi, A. Simchi: Mater. Sci. Eng. A, 2013, </p><p>449 vol. 585, pp. 222-232.</p><p>450 [58] C. Sun, M. Song, Z. Wang, Y. He: J. Mater. Eng. Perform., 2011, vol. 20, pp. 1606-</p><p>451 1612.</p><p>452 [59] B. Ogel, R. Gurbuz: Mater. Sci. Eng. A, 2001, vol. 301, pp. 213-220.</p><p>453</p><p>454</p><p>455</p><p>456</p><p>457</p><p>458 20</p><p>459 Table captions:</p><p>460 Table 1. Chemical composition of AA1050 sheets.</p><p>461 Table 2. Summary of AMCs strength from literatures.</p><p>462 Table 3. Contribution of strengthening mechanisms and yield strength obtained by </p><p>463 theoretical models and experiment in Al/SiCp composites.</p><p>464</p><p>465 Figure captions:</p><p>466 Figure 1. Engineering stress-strain curves of annealed aluminum (Al), monolithic </p><p>467 aluminum (Al-APB) and Al/10 vol.% SiCp composite produced by APB process. </p><p>468 Figure 2. STEM micrographs of Al/SiCp composite after different APB cycles; (a) 2,</p><p>469 (b) 5, (c) 7 and (d) 10 and (e) 14 cycles.</p><p>470 Figure 3. STEM micrograph of aluminum/SiCp interface.</p><p>471 Figure 4. TEM micrographs of aluminum after one cycle of APB process; (a) </p><p>472 surface (b) center of specimen.</p><p>473 Figure 5. TEM micrographs of Al/SiCp interface after 14 cycle of APB process.</p><p>474 Figure 6. Al/SiCp composite after 14 APB cycle: (a) EBSD/OIM and (b) grain </p><p>475 boundary maps.</p><p>476 Figure 7. FE-SEM micrographs of Al/SiCp composite after (a) 1, (b) 3, (c) 5 and (d)</p><p>477 10 and (e) 14 APB cycles.</p><p>478 Figure 8. (a) SEM micrograph of Al/SiCp composite along with its (b) aluminum, (c)</p><p>479 silicon and (d) carbon X-ray maps. EDS analysis of points (e) 1 and (f) 2.</p><p>480 Figure 9. X-ray diffraction (XRD) pattern of Al/SiCp composite. </p><p>481 21</p><p>482</p><p>483 Tables:</p><p>484 Table 1. Chemical composition of AA1050 sheets.</p><p>Element Al Si Fe Mn Cu Mg Zn Ti wt.% Bal. 0.2 0.22 0.02 0.01 0.01 0.01 0.01 485</p><p>486 22</p><p>487</p><p>488 Table 2. Summary of AMCs strength from literatures. AMCs Methods Reinforceme YS (MPa) UTS (MPa) Reference nt particle size</p><p>Al/5 wt.% Al2O3 Casting 20 µm ~112 ~157 [54]</p><p>Al/5 vol.% SiCp Casting 8 µm ~80 ~115 [55]</p><p>Al/3 wt.% Al2O3 Casting 50 nm ~107 ~162 [54]</p><p>Al/20 vol.% Al2O3 Casting+extrusion 12 µm ~175 ~220 [56]</p><p>Al/2 vol.% SiCp Friction stir welding 15 nm ~130 ~108 [57]</p><p>Al/20 vol.% SiCp Powder metallurgy 17 µm ~87 ~107 [58]</p><p>Al-5Cu/13vol.% SiCp Powder metallurgy 10 µm ~134 ~175 [59]</p><p>Al/10vol.% SiCp Accumulative press 10 µm 180 222 Present work bonding 489</p><p>490 23</p><p>491 Table 3. Contribution of strengthening mechanisms and yield strength obtained by</p><p>492 theoretical models and experiment in Al/SiCp composites.</p><p>Strengthening mechanisms and yield Al/SiCp micro-composite Al/SiCp nano-composite strength</p><p>Grain boundary () 64.9 75.6 Thermal mismatch () 6.8 39.6 Elastic mismatch () 5.4 34.4 Strain hardening () 49 42 Orowan looping () 2.4 172 Load-bearing () 1.5 0.3 Experimental yield strength () 148 210 Calculated arithmetic yield strength () 159 393 Calculated quadratic yield strength () 111 228 144 Calculated compounding yield strength () 289</p><p>493</p><p>494</p>
Details
-
File Typepdf
-
Upload Time-
-
Content LanguagesEnglish
-
Upload UserAnonymous/Not logged-in
-
File Pages23 Page
-
File Size-