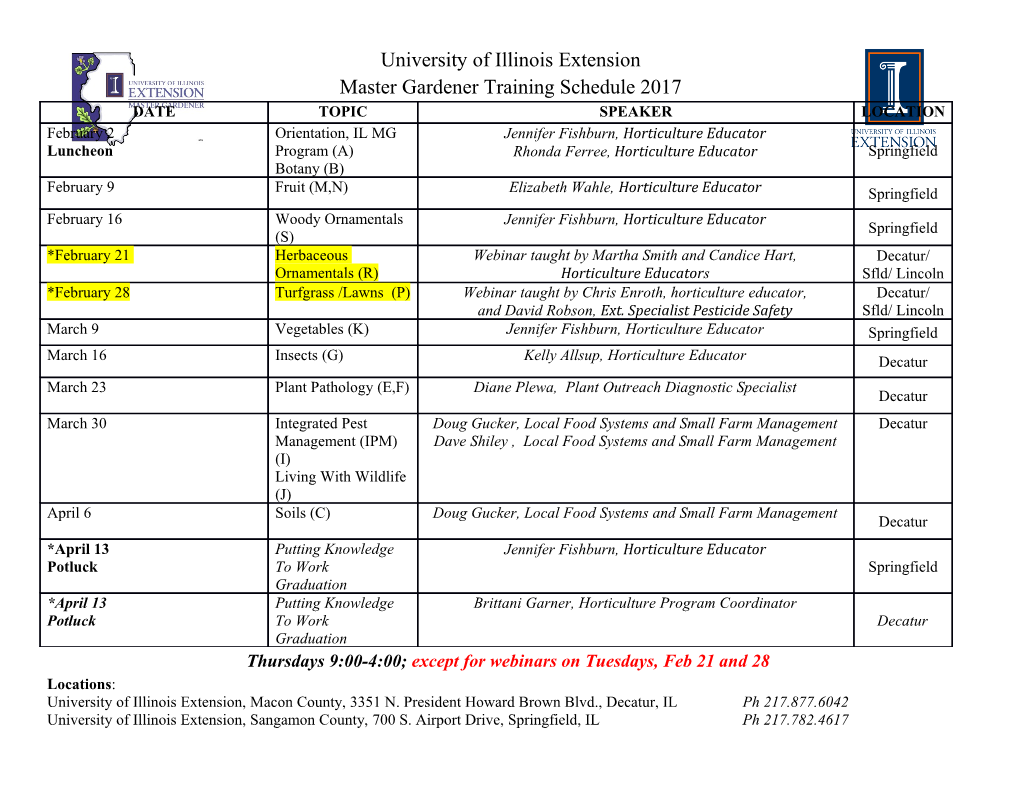
<p>[Version 3.0. Final draft. Last updated by Paul Falkowski 13 December 1999]</p><p>Integrated understanding of the global carbon cycle: A test of our knowledge</p><p>IGBP Carbon Working Group1</p><p>We are just beginning to understand how Earth's complex systems operate to sustain life on this planet. Despite our rudimentary knowledge, the scientific community is expected to provide reliable models to predict safe limits for human intervention, while simultaneously the effects of human activities on global systems is unprecedented. The traditional, disciplinary approach to understanding the effects of human activities on global systems cannot provide the requisite, reliable, prognostic Earth system models that are essential for rational policy formulation. While climatology, oceanography, geochemistry, and terrestrial and marine ecology all provide essential information about how the Earth functions, the ensemble of information is insufficient unless these discrete facets of the global system can be mechanistically linked. A truly interdisciplinary research effort is needed to develop even a minimal integrated model. As a step towards </p><p>1 Co-chairs and lead authors: P. Falkowski ([email protected]) and RJ Scholes ([email protected]); Members: E. Boyle, J. Canadell, D. Canfield, J. Elser, N. Gruber, K. Hibbard, P. Högberg, S. Linder, F.T. Mackenzie, B. Moore III, T. Pederson, Y. Rosenthal, S. Seitzinger, V. Smetacek, W. Steffen</p><p>1 that goal, the authors of this article met in Stockholm in October 1999 to explore how the known links between the carbon and nutrient cycles serve to integrate the terrestrial, freshwater, atmospheric, and oceanic components of the global system. We present here a conceptual framework to guide the synthesis of current knowledge and to inform the debate on international management of the carbon cycle. </p><p>Biogeochemical feedback and control mechanisms in the biosphere</p><p>Biological processes, most of which originated over 2 billion years ago, together with the geological and climatological processes with which they coevolved, determine the distribution of the elements of the biosphere {Schlesinger, 1997 #8616}. The biological assimilation, biochemical transformation, physical transport, and geological mobilization and sequestration of many elements are self-perpetuating and self- regenerating, leading to a ‘cycling’ of the elements. Like life itself, biogeochemical cycles are far from thermodynamic equilibrium. Rate-limiting reactions within key cycles have modified the tempo and mode of evolution, promoting biological diversity and ensuring the continuity of biogeochemical cycles throughout Earth's history. The resulting network of interlinked cycles is responsible for regulating the chemistry and climate of the ocean, atmosphere, and terrestrial ecosystems in the contemporary world. </p><p>Natural selection, acting on metabolic processes, imposes elemental requirements for life that are manifested at all scales from the organism to the whole planet. The carbon cycle is central to all life on Earth, since about half of all biomass consists of carbon, but the carbon cycle did not evolve in vacuo. It is coupled to the dynamics of the approximately 20 other elements necessary for life (Fig. 1). This coupling occurs at the biochemical and cellular level in organisms, and constrains the rates of processes and the sizes of storage pools of carbon. Consequently, carbon and nutrients cycle through ecosystems within a relatively narrow range of proportions. While there is variation in this ‘ecosystem stoichiometry’, the underlying causes of the variations are largely understood, and therefore predictable. </p><p>2 Like physiological processes of which they are a part, biogeochemical cycles form interdependent, self-regulating systems, replete with feedback controls and processes. The stoichiometry of bioessential elements becomes unbalanced when supply processes do not match demands, leading to growth limitation. The metabolic machinery for some biological processes, such as photosynthesis and aerobic respiration, is widely distributed across diverse aquatic and terrestrial organisms. In contrast, other key biogeochemical processes, such as biological nitrogen fixation (BNF) and nitrification, are found in a relatively small number of taxa. For example, most oceanic BNF is performed by a small subset of cyanobacteria and terrestrial BNF is carried out by a subset of heterotrophic bacteria. The paucity of nitrogen fixers in marine and terrestrial systems leads to a biogeochemical bottleneck that co-regulates photosynthetic carbon fixation in both ecosystems {Falkowski, 1997 #7973}. This bottleneck, in turn, affects the supply of organic carbon for heterotrophic respiration and BNF itself. This is a simple illustration of a negative feedback that helps drive global biogeochemical cycles towards a quasi-steady state. </p><p>On geologically short time scales, human activities have altered many biogeochemical processes to varying degrees, both spatially and temporally, resulting in unbalanced growth conditions relative to biological demand. As a result, biological assimilation of anthropogenic sources of CO2, N, and P are not necessarily coupled, potentially leading to deviations from steady state. How well can we predict the sign of the feedbacks or the outcome of these human activities?</p><p>While earth system scientists conceptually understand many of the key processes, we often fail to reproduce critical phenomena in simple models. As human experience has witnessed an infinitesimally small fraction of Earth's history, most of our knowledge is based on proxy data that are reconstructed to form (whenever possible) testable, consistent hypotheses. In some cases, essential information is obscured or absent in our </p><p>3 reconstruction of the geological record. For example, in spite of its importance, at present we do not fully understand the processes that led to the large increase in atmospheric O2 approximately 2.2 billion years ago {Holland, 1998 #8620}. The sequestration of organic carbon in the lithosphere is a corollary of the net accumulation of atmospheric O2 by oxygenic photosynthesis. Sequestration requires an imbalance between photosynthesis and respiration; there is no clear consensus on the factors that led to such conditions on a planetary scale. The rapid warming of Earth's atmosphere ca. </p><p>55 million years ago appears to correspond with a rapid rise in atmospheric CO2 and CH4. The causes of the rise in these gases are unclear, but the implication is that carbon ‘sequestered’ in one or more reservoirs (e.g. the ocean or lithosphere) was mobilized and partially transferred to the atmosphere (Katz et al 1999).</p><p>Control of the earth system over the past 400,000 years</p><p>There is much more geological and geochemical information about Earth's variability during the past million years, yet our (disciplinary) approach to elucidating processes has been unsatisfactory. A clear example is our lack of understanding of the causes of glacial-interglacial variations in climate and atmospheric CO2. Analyses of air trapped in Antarctic ice reveal that during the past 420,000 years the atmospheric CO2 has varied in concert with climate cycles, alternating between 180-200 ppmv at the peak of glacial periods and 265-280 ppmv during the warm interglacials {Petit, 1999 #8622} . Although the timing of these climate cycles show remarkable correspondence to cyclic variations in Earth's orbital parameters with typical frequencies of 21, 41 and 100 kyr, ( the so-called ‘Milankovich cycles’; Hays et al. 1976; Fig. 2), the direct radiative effects of the changes in energy received from the sun are insufficient to drive glacial- interglacial climate cycles (Hansen et al 1984). The strong coupling between atmospheric</p><p>CO2 and temperature suggests that atmospheric CO2 may be the primary amplifier of </p><p>4 climate change during glacial terminations, a hypothesis originally proposed by Arrhenius (1896). </p><p>[insert Figure 2: the 400 kY CO2 record and its spectral analysis – ]</p><p>The glacial-interglacial transitions are an example of a highly reproducible ‘flip-flop’ between two quasi-stable states {Smith, 1999 #8621}. Any mechanism(s) invoked to account for glacial-interglacial transitions must explain the following gross features of the atmospheric CO2 signal: the periodicity of the cycles; the transfer of carbon between terrestrial and oceanic pools (as revealed by changes in 13C content); the remarkable consistency of the upper and lower limits; the apparent fine control for a period of many thousands of years around those limits; the relatively rapid transition from glacial to </p><p>5 interglacial; and the initially steep, but punctuated and eventually gradual transition into the glacial. </p><p>The upper and lower limits of atmospheric CO2 attained in the glacial and interglacial periods respectively, contain information about the control systems and their feedbacks. We propose that the lower limit is likely to reflect terrestrial ecosystem control and the upper limit primarily oceanic control. Nutrient-mediated interactions between land and ocean transfer the control from one to the other on a periodic basis. We speculate that widespread terrestrial ecosystem failure below 180 ppmv CO2 establishes an active negative feedback control loop. This concentration of CO2 is an ‘ecosystem compensation point’ – the concentration where the photosynthetic assimilation of CO2 is balanced by autotrophic respiration (note: this compensation point is primarily dictated by C3 plant ecosystems). In the dry climate of the glacial period, water use efficiency, which is strongly influenced by CO2 concentration, would be a particularly important mechanism. We suggest that the upper (280 ppmv) CO2 concentration limit is an upper equilibration point between the atmosphere and the oceans, perhaps resulting from the bottleneck imposed on biospheric carbon fixation by nitrogen fixation, compounded by a loss of dissolved inorganic nitrogen due to enhanced denitrification {Codispoti, 1995 #8090; Falkowski, 1998 #8136}. What causes the terrestrial system to switch between these two quasi-stable domains? The onset of glacial periods is systematically keyed to decreased solar radiation due to Milankovich forcing {Imbrie, 1992 #3940}. The direct radiative forcing is amplified by a reorganization of oceanic circulation, increased sea ice cover in the Southern Ocean, and a decrease in the hydrological cycle. Positive feedbacks, through the a reduction in greenhouse gases such as H2O, CO2 , and CH4, and the increased planetary albedo accompanying the formation of continental and sea ice, would further cool the Earth. Superimposed on these processes is an increase in aeolian iron, which stimulates oceanic primary productivity {Martin, 1990 #5774}and as well as oceanic nitrogen fixation {Falkowski, 1997 #7973}. Declining biotic activity on land, combined with cooling and drying, eventually reduces the flux of nutrients from land to sea to the </p><p>6 point where further net uptake of carbon by the oceans balances the oceanic emissions, and control is primarily through the effect of atmospheric CO2 on terrestrial carbon balance. The glacial terminations are also tied to externally imposed radiative forcing, but are of opposite sign. Enhanced warming leads to an initial outgassing of oceanic </p><p>CO2 and a stimulated hydrological cycle that corresponds with a marked decline in aeolian mineral fluxes { {Martin, 1990 #5774; Petit, 1999 #8622; Broecker, 1998 #8222}. Retreating ice sheets and the corresponding flooding of continental margins accelerates denitrification, while the simulatenous decline in aeolian iron would lead to a reduction in oceanic productivity and nitrogen fixation. Terrestrial biological activity, stimulated by increased the moisture and warmth, accelerates the mobilization of soluble nutrients such as P, and Si from the geosphere. These elements initially build up in soils and terrestrial biomass, and then flow into coastal ecosystems via rivers. The flux of nutrients from the land stimulates oceanic net primary production and burial, which begins to stabilize and, subsequently, upon an external forcing set by a Milankovich cycle, draw down atmospheric CO2. Our simple analysis suggests that the glacial-interglacial transitions reflect a nutrient-driven control and feedback of the carbon cycle involving terrestrial, oceanic and atmospheric realms. The ‘normal’ state of the biosphere during the past 400,000 years is glacial, with low atmospheric CO2 and resultant terrestrial vegetation stress. There is no historical evidence for a stable state or domain of attraction substantially above 280 ppmv. The point of our speculation is not to provide the ‘answer’ for the causes of the glacial-interglacial transitions, but rather to illustrate how an interdisciplinary, systems-based approach can generate a rich set of mechanisms to address a problem which has eluded disciplinary solutions.</p><p>7 Disturbance of global biogeochemistry during the past 200 years</p><p>There is even more abundant and less ambiguous biogeochemical data for the period of observational record, but prognostic models, based on a disciplinary approach, are not necessarily more accurate. For example, we know that global biogeochemical cycles of all the bioessential elements have been significantly altered due to human activities within the past 200 years (Table 1). CO2 released by the burning of fossil fuels and from changes in land use have increased atmospheric CO2 concentrations from the interglacial ‘setpoint’ of ca. 280 ppmv to more than 370 ppmv at present. Not only is this concentration is unprecedented over the last 420,000 years of Earth's history, but the rate of increase in atmospheric CO2 is about two orders of magnitude lower than that resulting from human activities since the Industrial Revolution (IPCC, 1995). </p><p>The production of synthetic fertilizers, the cultivation of nitrogen-fixing crops, and deposition of fossil fuel-associated nitrogen are together of the same order of magnitude as natural BNF, thus doubling the nitrogen inputs to the biosphere (Galloway et al. 1996). Mining of phosphorus for fertilizer use has increased P inputs to the biosphere by approximately four-fold. </p><p>The cycling of these elements is normally kept in proportion through the production and subsequent oxidation of organic matter in the biosphere. There are large uncertainties in how alterations in N and P cycles of this magnitude and rapidity interact with human perturbations to the global C cycle. Most global models deal with one element, thus ignoring the potential linkages and feedbacks among the others. At first glance, one might conclude that the simultaneous increases in N fixation and P production would stimulate the biological sequestration of carbon in terrestrial and </p><p>8 marine ecosystems. Will such stimulation provide salvation from the continued emissions of CO2 to the atmosphere as a consequence of human activities?</p><p>Several models have computed the partitioning of anthropogenic CO2 from 1850 to 1990 (Sarmiento et al. 1992; Bruno and Joos 1997; Ver et al. 1999). The results of one such model suggest the existence, for most of this century, of a modest oceanic CO2 sink and a larger terrestrial carbon sink on non-agricultural land (Fig. 3). Without these sinks, the rate of increase in atmospheric CO2 would be twice the observed rate. While it is likely that both sinks will continue to exist and grow over the next few decades, there is increasing evidence for a leveling-off, and even a decline in the strength of these sinks within the next 50 to100 years. </p><p>Biotic sinks for CO2 require nutrients for the formation of organic matter. Enhanced net primary production in forests associated with enhanced inputs of N from atmospheric deposition has been suggested as an important sink for anthropogenic CO2 (Schimel 1995). The magnitude of this sink is highly uncertain and depends on a number of factors including the C:N ratio of the stored organic matter and the degree of N- saturation of soils (Nadelhoffer et al. 1999). While N deposition is predicted to continue, and has the potential to enhance the carbon sink in N-limited ecosystems, it will become decreasingly effective at doing so. Future N deposition will largely occur on already N- saturated soils, such as in Western Europe forests, China and India, and on agricultural lands in the tropics whose capacity to sequester C is intrinsically small and where soils are mostly P-limited (Hal and Matson 1999). </p><p>Enhanced terrestrial productivity in response to increased levels of atmospheric </p><p>CO2 has also been proposed as a significant terrestrial sink (Schimel 1995). The relationship between terrestrial Net Primary Production (NPP) and atmospheric CO2 reaches a plateau at high atmospheric CO2. Some experimental evidence suggests that in natural ecosystems it may level off at 10-20% above current terrestrial NPP, at an </p><p>9 atmospheric CO2 level as low as double pre-industrial concentrations (i.e. 550-650 ppm), because of negative feedbacks such as nutrient limitation (ref: Christiaan Korner). In addition, the associated increased temperature will bring higher respiration, particularly by soil microbes, which may completely counteract or even overtake the CO2 uptake. This may in some ecosystems change current C sinks into C sources. The magnitude and and rate of this change is, however, uncertain because plants can acclimate to changes in temperature (Dewar et al. 1999) and functional and compositional shifts may take place in microbial communities (Zogg et al. 1997). In addition to these physiological considerations, land use change plays a major role in the strength of the carbon source/sink dynamics. Forest conversion to pasture and agriculture, in order to sustain increased food and fiber demands largely in the developing world, simultaneously provides additional carbon sources and reduces the area available for active sinks. Abandonment of agricultural land and regrowth of forests, largely in the temperate northern hemisphere, is likely a significant terrestrial CO2 sink at present, but cannot be sustained indefinately. </p><p>Oceanic net primary production is not directly affected by atmospheric CO2, so no substantial increase in the strength of the biological pump is anticipated as a direct consequence of CO2 emissions. For the oceans to become a major ink in the future, one or more of the following conditions must be met: limiting nutrients must be added to the ocean; nutrients that are unused in the Southern ocean must be consumed; or the elemental composition of the organic matter must change (for instance, the ratio of calcite to organic matter in the sinking flux could decrease) {Sarmiento, 1992 #4915}. </p><p>The oceanic sinks of carbon are both abiotic and biotically controlled, and the interactions between the two control processes are poorly understood. The present uptake of anthropogenic CO2 by the oceans is thought to be primarily a consequence of abiotic processes, controlled by the transport of dissolved inorganic carbon from the surface to </p><p>10 the deep oceans through circulation. Coupled climate-ocean simulations (Manabe and </p><p>Stouffer; Mitchell et al.) suggest that CO2-induced global warming may lead to increased vertical stratification of the water column. Should this occur, the physical transport of carbon from the upper ocean to the deep ocean will be reduced with a resulting decrease in the rate of sequestration of atmospheric carbon in the ocean (Sarmiento et al., 1998; Joos et al. 1999). </p><p>The same physical processes that lead to increased stratification will conspire to reduce the efficacy of biological carbon sequestration in the ocean. </p><p>Although nutrient loading has resulted in coastal eutrophication on a global scale, virtually all the fixed nitrogen is blocked before it reaches the ocean, through through denitrification and burial in near coastal environments (Christensen et al. 1987; Seitzinger and Giblin 1996). Coastal denitrification effectively decouples the terrestrial and oceanic N cycles. No carbon is stored during denitrification and the burial of C in coastal organic matter associated with enhanced N inputs is small relative to other C sinks. The transport of iron from land to sea via wind-borne dust is critical to support primary production and nitrogen fixation in the ocean. Iron limitation of photosynthetic carbon fixation in the open ocean has been experimentally demonstrated ({Coale, 1996 #8114}. While there appears to be a remarkable correspondence between aeolian fluxes of iron and other minerals and atmospheric CO2 concentrations between glacial and interglacial periods, controls on this flux are poorly understood. To the extent that aridity affects aeolian iron supplies, iron fluxes to the ocean are likely to decrease as the hydrological cycle becomes more active in the coming decades {Dai, 1997 #8146}. </p><p>11 This analysis of the effects of nutrients on the carbon cycle is by no means complete or comprehensive. Rather, we have attempted to demonstrate the complexity of the issues, and our understanding of the limits of natural remediation. There is no natural ‘savior’, waiting to assimilate all the anthropogenic CO2. It is difficult to conceive how human geoengineering efforts could effectively sequester anthropogenic </p><p>CO2 on the scale necessary to compete with emissions. No one can predict with certainty the planetary outcome of human activities in the decades to come, but over the past decade we have witnessed the birth of interdisciplinary Earth system science. Unless we learn how to raise that approach to a mature level, we will continue to wallow in uncertainty in areas critical to both human civilization and the biota with which we share the planet. </p><p>Acknowledgements</p><p>The workshop was organized by the International Biosphere-Geosphere Programme and the Royal Swedish Academy of Science, in collaboration with Stockholm University and the Swedish University of Agricultural Sciences. Financial support was provides by the Swedish Millenium committee and MISTRA (Swedish Foundation for Strategic Environmental Research). The workshop was the first of five Stockholm workshops contributing to the IGBP synthesis project. Thanks to John Raven for comments.</p><p>12 Tables Table 1. Examples of human intervention in the global biogeochemical cycles of C, N, P, S, water, and sediments. Data are for the mid-1900s.(1)</p><p>Element Flux Magnitude of Flux in MTY-1 Interference(2) Natural Anthropogenic Factor %</p><p>Terrestrial respiration 61000 Humans have affected as much as 40% of /decay CO2 NPP on land and several percent of marine NPP. Carbon-containing compounds of C Fossil fuel and land use biological origin are the major terrestrial and 8000 13 CO2 marine N store, and constitute important stores of P and S. C strongly influences the rate of extraction of P from the geosphere and supplies the energy for biological N fixation from the atmosphere. CO are important greenhouse gases. The interference factor for CH the earth’s surface to the atmosphere is roughly 50%. Natural biological fixation 130 Anthropogenic N constitutes about one-half of the annual river and atmospheric Fixation owing to rice discharge of total N to the ocean. Much of cultivation, combustion of the anthropogenic fixed N on land is N fossil fuels and production 108 currently unaccounted for in the balance for of fertilizer 140 N on land. N appears to be the most widespread limiting nutrient in terrestrial and aquatic systems. Reactive N from anthropogenic activities can initially lead to carbon storage on land but also can promote acidification and shifts in the species composition of terrestrial ecosystems. Antropogenically derived N can lead to eutrophication of aquatic systems. Chemical weathering 3 Anthropogenic P constitutes about one-half of the annual river flux of total P to the P Mining 12 400 ocean. The land is presently accumulating P from application of fertilizer to croplands and other human activities. P can be limiting in tropical, terrestrial ecosystems and in some marine systems. It is the cause of eutrophication in certain aquatic systems. Natural emissions to 80 Anthropogenic S emissions are equivalent to atmosphere at earth’s about 70% of the S rained out of the </p><p>13 surface atmosphere each year. S is occasionally S 113 limiting in agricultural systems. Excessive Fossil fuel and biomass 90 deposition of industrial and transportation S burning emissions acidifies soils and freshwater, and potentially can reduce NPP and C storage. Volcanic and anthropogenic sulfate aerosol can lead to a cooling of the climate. O, H Precipitation over land 111x1012 Water vapor is the most important </p><p>(as H2O) 12 greenhouse gas and the most important Global water usage 18x10 16 agent for the transport of nutrients about Earth’s surface. Water can control the rates of soil-forming processes and the duration of the growing season in arid areas. The transfer of water between ice and the ocean on interglacial/glacial time scales controls the area of the land surface, and to some extent the global albedo and the intensity of the thermohaline circulation of the ocean. Humanity now uses about 55% of accessible water runoff from the continents, and there is considerable concern for the future of water quality and usage for some parts of the world. Long-term pre-industrial 1x1010 The 40,000 dams on the world’s rivers have river suspended load significantly affected water and sediment discharge to the ocean. However there is a 200 Sediments Modern river suspended 10 lack of conclusive evidence whether or not load 2x10 dams have substantially reduced the amount of these materials reaching coastal marine environments in modern times.</p><p>(1) Updated from Mackenzie, 1993; (2) Interference factor (%) = (anthropogenic emissions/natural emissions)x100.</p><p>14 15 16 Figure Legends</p><p>Figure 1. The cycles of carbon, nitrogen, phosphorus and several other biologically essential elements are closely coupled in terrestrial, freshwater, and marine ecosystems. These three subsystems of the biosphere are in turn linked to each other via transport through the hydrological cycle and the atmosphere. Ocean-atmosphere gas exchange, which is driven by marine biology in the long term, largely establishes the atmospheric concentration of CO2 and thus the global climate. Terrestrial plant physiology is very sensitive to climate and the concentration of CO2 in the atmosphere. The state of the vegetation controls the rate of transfer from the land to the sea of elements essential to marine organisms, thus closing the control loop.</p><p>Figure 2. Upper Panel: The atmospheric concentration of CO2 over the past 400,000 years can be determined from the gases trapped in polar ice such as drilled at Vostok, Antarctica (Petit et al. 1999). This record spans four glacial/interglacial cycles, whose period is keyed to the subtle variations in solar radiation received by the earth (the Milankovich cycles) caused by variations in the orbital parameters of the earth. The atmospheric CO2 shows remarkable consistency in its lower and upper bounds, at 180 and 280 ppmv respectively, suggesting control feedback mechanisms at those levels. In the last two centuries, land transformations and the combustion of coal, oil, and natural gas have taken the atmospheric CO2 far above the former upper bound, and are projected to continue to do so. The global system has not experienced such a high [CO2]atm, nor such a rapid rate of change, for millions of years. Lower Panel: The magnitude of variability in atmospheric CO2 over a range of time-scales going from billions of years to hours shows that the anthropogenic perturbation is indeed a remarkable event in the history of earth. Atmospheric CO2 variations of the magnitude that are projected for the next century very likely have not been seen on earth for the last 10 million years (Berner, 1983).</p><p>17 Figure 3. As a result of the rapid forcing of the atmospheric CO2 over the past two centuries, the global ecosystem is in disequilibrium. The mean annual increase in atmospheric CO2 currently accounts for about half of the CO2 transferred to the atmosphere by human activities; the rest is taken up by the biosphere. Over a period of several hundred centuries, almost all of it will be absorbed by the oceans. In the immediate term most is converted to increased biomass and soil carbon in terrestrial ecosystems. These ‘terrestrial sinks’ must be finite, but their capacity is unknown, and their physical location, controlling processes and dynamics over the next century are controversial.</p><p>18 References</p><p>[Iron fertilization in oceans]</p><p>[280ppm is equilibrium point of ocean-atmosphere models – Berrian Moore]</p><p>Delwiche, CC GE Likens 1977 Biological response to fossil fuel combustion products. In Stumm W (ed) Global chemical cycles and their alteration by man. Dahlem Konferenzen, Berlin 73-88.</p><p>Elser, JJ, DR Dobberfuhl, NA MacKay, JH Schampel. Organism size, life history and N:P stoichiometry. BioScience 46, 674-684.</p><p>Falkowski, P. (1997). “Evolution of the nitrogen cycle and its influence on the biological </p><p> sequestration of CO2 in the ocean.” Nature 387: 272-275.</p><p>Hansen, J et al 1984 Climate sensitivity: analysis of feedback mechanisms. In: Climate processes and climate sensitivity ed. J Hansen and T Takahashi. Am. Geophys. Union Mon. 29, 130-163</p><p>Katz and Miller</p><p>Korner, Christiaan [leveling off of CO2/NPP relationship]</p><p>Likens, GE, HF Bormann, NM Johnson 1981 Interactions between major biogeochemical cycles in terrestrial ecosystems. In Likens, GE Some perspectives of the major biogeochemical cycles. Wiley, NY pp 93-112. SCOPE 17</p><p>19 Melillo, J.M., Callaghan, T.V., Woodward, F.I., Salati, E. & Sinha, S.K. (1990). Effects on Ecosystems. In Climate Change. The IPCC Scientific Assessment, eds. J.T. Houghton, G.J. Jenkins, & J.J. Ephraums, pp. 283-310. Cambridge University Press.</p><p>Sarmiento, J.L., T. M. C. Hughes, R. J. Stouffer and S. Manabe, 1998. Simulated response of the ocean carbon cycle to anthropogenic climate warming, Nature, 393, 245-249.</p><p>Schimel, D.S. 1995. Terrestrial ecosystems and the carbon balance. Global Change Biology 1: 77-91.</p><p>Steffen, W., Noble, I., Canadell, J., Apps, M., Schulze, E.-D., Jarvis, P.G., Baldocchi, D., Cias, P., Cramer, W., Ehleringer, J., Farquhar, G., Field, C.B., Ghazi, A., Gifford, R., Heimann, M., Houghton, R., Kabat, P., Körner, Ch., Lambin, E., Linder, S., Mooney, H.A., Murdiyarso, D., Post, W.M., Prentice, C., Raupach, M.R., Schimel, D.S., Shvidenko, A. & Valentini, R. 1998. The terrestrial carbon cycle: Implications for the Kyoto protocol. Science, 280: 1393-1394.</p><p>Schlesinger, W. H. (1997). Biogeochemistry: An Analysis of Global Change. New York, Academic Press.</p><p>[potential referees: Bert Bolin, Dave Schimel, Martin Heimann, Jim Galloway, Inez Fung, Jim Orr, Tony Janetos, Fortunat Joos, Jorge Sarmiento]</p><p>20 21</p>
Details
-
File Typepdf
-
Upload Time-
-
Content LanguagesEnglish
-
Upload UserAnonymous/Not logged-in
-
File Pages21 Page
-
File Size-