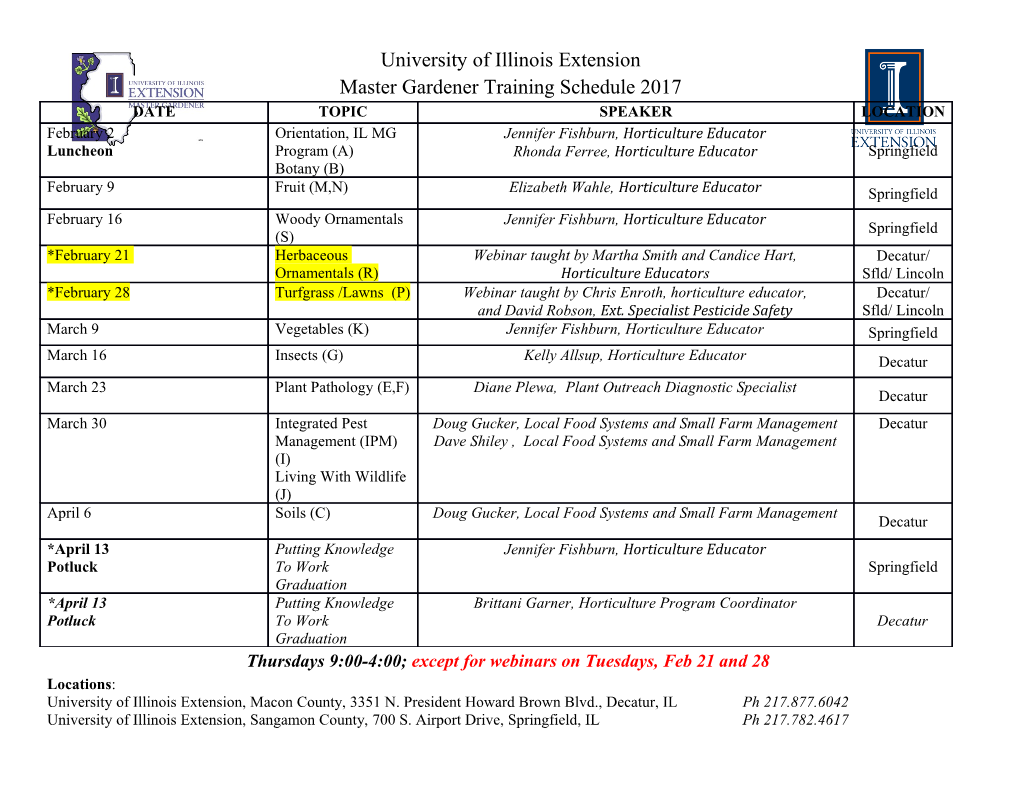
<p> 0a3c5a2395ed61be11492a7dbf31b8fe.doc 2018/1/9</p><p>Published online 10 October 2007 | Nature 449, 652-655 (2007) | doi:10.1038/449652a </p><p>News Feature Biofuel: The little shrub that could - maybe</p><p>India, like many countries, has high hopes for jatropha as a biofuel source, but little is known about how to make it a successful crop. Daemon Fairless digs for the roots of a new enthusiasm.</p><p>Daemon Fairless </p><p>STRINGER INDIA/REUTERS With a top speed of about 110 kilometres an hour, India's Shatabdi Express is not much to brag about by the standards of a French TGV or a Japanese Shinkansen train. Nonetheless, as the stock for one of the country's fastest and most luxurious passenger lines, the Shatabdi trains have a certain prestige. So when, on New Year's Eve 2002, the Shatabdi train from New Delhi to Amritsar was powered in part with biodiesel for the first time, it was a clear statement of the government's desire to wean India off imported petroleum.</p><p>Diesel is India's main liquid fuel: the country burns roughly 44 million tonnes, or 320 million barrels, of the stuff a year, as opposed to about 94 million barrels of gasoline. The trains account for a significant part of that. Kunj Mittal, who heads the government-operated rail service's engineering and traction division, says its fleet of 4,000 engines currently burns about 1.7 million tonnes a year, and that he wants to replace at least 10% of that with biodiesel at some unspecified point in the future. But he would need 200 million litres of biodiesel a year. Which is a problem. “At this stage,” says Mittal, “there is no mass production of biodiesel.”</p><p>Like many others around India, the rail service is looking to an unprepossessing, poisonous scrub weed to try to do something about that. It has planted a million Jatropha curcas seedlings on unused land along its tracks and elsewhere. It's just one symptom of the jatropha fever that is spreading around the country and the world — to the slight bewilderment of some of the scientists who best understand the shrub.</p><p>Jatropha, a member of the euphorbia family, originated in Central America. It has long been used around the world as a source of lamp oil and soap, and also as a hedging plant. One of its great selling points as a biofuel is the fact that growing it need not compete with the cultivation of food. Of 306 million hectares of land considered in a report by India's Ministry of Rural Development, 173 million are already under cultivation but the rest is classified as either eroded farmland or non-arable wasteland. That's the sort of land that jatropha can thrive on, with bushes living up to 50 years, fruiting annually for more than 30 years and weathering droughts with aplomb1. In the early 2000s then-president A. P. J. </p><p>1/53 0a3c5a2395ed61be11492a7dbf31b8fe.doc 2018/1/9</p><p>Abdul Kalam repeatedly endorsed the plant for its potential contributions to energy security and as a route to greening barren land. Jatropha has been held to promise a reliable source of income for India's poor rural farmers and energy self- sufficiency for small communities — all while reducing fossil-fuel greenhouse-gas emissions and soil erosion.</p><p>Oasis in the desert: jatropha cultivation can halt soil erosion, increase water storage in the soil and transform barren expanses into lush, productive land.J. CHIKARA In 2003, India's Planning Commission recommended a national mission on biofuel, a two-phase project for wide-spread cultivation of jatropha on wasteland across much of India. The first phase of the mission aims for 500,000 hectares of jatropha grown on government land across the country. The biodiesel would be produced primarily by panchayats — local governing bodies — at the village level, coordinated at the national level by a consortium of government departments. Should the first phase go according to plan, India's central government would embark on the second phase of the mission — planting a total of 12 million hectares of the plant and privatizing the production of jatropha biodiesel.</p><p>Although it seems likely to go ahead eventually, various ministerial meetings that might have given the national mission on biofuel the seal of approval have been postponed in favour of higher-priority issues. Despite this, several states have enthusiastically hopped aboard the jatropha express, providing free plants to small-scale farmers, encouraging private investment in jatropha plantations and setting up biodiesel processing plants. The Ministry of Rural Development, which is set to coordinate the national mission on biofuel when it is approved, estimates that there are already between 500,000 and 600,000 hectares of jatropha growing across the country.</p><p>And India is not alone in its hopes for the shrub. In February 2007 China, which claims to have 2 million hectares of jatropha already under cultivation, announced plans to plant an additional 11 million hectares across its southern states by 2010. Neighbouring Myanmar (Burma) has plans to plant several million hectares; and the Philippines, as well as several African countries, have initiated large-scale plantations of their own. India looks forward to encouraging more such schemes and quite possibly profiting from them. “Once we have an operational programme and have something to offer the world,” says Krishna Chopra, the recently retired principal adviser to India's Ministry of New and Renewable Energy, “I think exporting the know-how would certainly be one of the first areas to develop.” </p><p>The great unknown</p><p>Although there is reason to be enthusiastic about jatropha's potential as a biodiesel feedstock in India and beyond, there is one rather sobering concern: despite the fact that jatropha grows abundantly in the wild, it has never really been domesticated. Its yield is not predictable; the conditions that best suit its growth are not well defined and the potential environmental impacts of large- scale cultivation are not understood at all. “Without understanding the basic agronomics, a premature push to cultivate jatropha could lead to very unproductive agriculture,” says Pushpito Ghosh, who has been working on the plant for the best part of a decade, and who is now director of the Central Salt and Marine Chemicals Research Institute (CSMCRI) in Bhavnagar.</p><p>2/53 0a3c5a2395ed61be11492a7dbf31b8fe.doc 2018/1/9</p><p>When Ghosh first arrived at the CSMCRI, the United Nations Development Programme (UNDP) had already given the institute funding for the cultivation of a modest jatropha plantation, although not for biofuels work. The idea was to see “how to make use of waste land, coastal areas and sand dunes”, Ghosh says.</p><p>The plantation started off as an unirrigated, unfertilized, 20-hectare patch of exhausted scrub: Ghosh wasn't particularly impressed when he first saw it. “There were shrubs and they were growing,” he recalls, “but it didn't look to me that it had what was required to make a successful plantation. 'Where are the seeds?' I said to myself. I didn't see too many of them. Merely planting and letting jatropha grow doesn't necessarily lead to productive growth.” Nonetheless, the fact that jatropha lived up to its reputation as a shrub that could eke out a living on relatively barren land piqued the interest of India's Department of Biotechnology, which provided a little further funding for exploration of biofuel possibilities using cuttings from three of the most productive plants in the UNDP trial.</p><p>The seedlings were planted in small plots spread over patches of degraded, untended land in the eastern state of Orrisa. “The results were not outstanding,” says Ghosh, “but they were consistent.” Several plants yielded around 1.5 kilograms of seed, enough for about 0.4 litres of diesel. As modest as the results were, says Ghosh, they created a lot of interest. “For the first time,” he says, “we were doing something in a systematic way.”</p><p>The CSMCRI's work also caught the imagination of Klaus Becker, who arrived at the institute in 2000 as a visiting agricultural scientist from the University of Hohenheim in Germany. The original UNDP plot inspired him far more than it had the sanguine, measured Ghosh. “I saw all this green in what is otherwise a complete desert. There was absolutely nothing else around it. 'Look,' I told Ghosh, 'if you get this working, you'll be the first in the world'.” </p><p>From seed to oil</p><p>Becker returned to Germany and set about fund-raising. By 2003 he had cobbled together a €1.7-million (US$1.9-million) research fund comprised of grants from DaimlerChrysler, the German Investment and Development Company in Cologne, India's Council of Scientific and Industrial Research and the University of Hohenheim. With these funds, Ghosh and his team — working in collaboration with Becker and scientists at DaimlerChrysler — began exploring the transesterification needed to turn jatropha into biodiesel. The process had already been established by Nicaraguan researchers during the 1990s2 and it wasn't long before Ghosh and his team were producing small batches.</p><p>“You could tell simply by looking at it that it was fairly good quality,” says Ghosh of their first attempts. Chemists at DaimlerChrysler's Stuttgart labs analysed it in more detail than the CSMCRI was able to and judged it easily good enough to meet European standards. Further tests at the Austrian Biofuels Institute (ABI), which pitted the CSMCRI's jatropha biodiesel against fuels from other feedstocks, showed that it “clearly outperformed biodiesel from rapeseed, sunflower and soya bean oil in [its lack of a propensity to oxidize],” says the ABI's Werner Körbitz, adding that the fuel “showed a fully satisfying performance concerning power, efficiency and emissions”.</p><p>Ghosh's vision — and part of the CSMCRI's mandate — was to create a version of this transesterification process that was both inexpensive and easily replicable at the village level. Nearly 80,000 of India's 600,000 villages currently have no access to fuel or electricity — in part because there isn't enough fuel for a fuel </p><p>3/53 0a3c5a2395ed61be11492a7dbf31b8fe.doc 2018/1/9 distribution network. “If people can grow oil directly in villages and produce biofuels themselves in decentralized plants,” says Ghosh, “then they can achieve energy self-sufficiency. My colleagues and I are deeply committed to this principle.”</p><p>“The constant urge to simplify and to ensure that every gram of jatropha is turned into something valuable was a tremendous motivator,” he says, looking back at the project. But while he and his colleagues were still congratulating themselves on a job well done, the Times of India ran a story announcing that DaimlerChrysler was set to test two of its Mercedes C-Class cars on a 6,000- kilometre road test across the length and breadth of India using the CSMCRI's jatropha biodiesel. </p><p>Up the Khardungla pass</p><p>It was the first Ghosh had heard of it. “Our focus all along has been biodiesel as a fuel for village folk,” he says, “not for fancy urban folk.” And on top of that there was an obvious practical difficulty. Up to this point, Ghosh and his team had only ever produced a few litres of it at a time: you can't get across India on that.</p><p>Within a few months, though, Ghosh's team had developed a transesterification unit capable of producing about 250 litres a day — adequate for use in villages and small-scale industry3. The Mercedes ran entirely on 100% jatropha biodiesel from this unit throughout April and May 2004 without any significant engine modifications. In the summer of 2005, DaimlerChrysler had several automotive journalists take the cars on a high-altitude test through the Himalayas, including Khardungla pass, which, at 5,359 metres above sea level, is one of the world's highest motorable roads.</p><p>Pushpito Ghosh tops up a vehicle that has covered 48,000 kilometres powered only by jatropha biodiesel.S. L. PUROHIT While Ghosh and his colleagues were making sure that jatropha could be processed as a reliable source of biodiesel, several of India's state governments were busy promoting their own jatropha cultivation campaigns. The state of Chhattisgarh, which has the most well-developed biodiesel programme in the country, has distributed 380 million jatropha seedlings to farmers, free of charge, over the past 3 years, enough to cover 150,000 hectares with the shrub. Shailendra Shukla, executive director of the Chhattisgarh Biofuel Development Authority (CDBA), says the state has also provided 80 oil presses to various village panchayats, and guarantees to buy back jatropha seeds — which have to be hand-picked off the shrubs — at 6.5 rupees (about US$0.16) per kilogram in order to stimulate confidence in the crop. Several local businesses have popped up across the state, says Shukla, that are now operating micro-refineries. “These are small businesses that provide biodiesel for the use in tractors, irrigation pumps, jeeps and village power generators.”</p><p>4/53 0a3c5a2395ed61be11492a7dbf31b8fe.doc 2018/1/9</p><p>Ghosh says that the CSMCRI has received an order for a refinery from the country's Defence Research and Development Organisation, part of India's Ministry of Defence. He explains that the unit would be capable of producing about 1,000 litres a day and would cost about 14 million rupees to install. In such a plant, he says, each litre of biodiesel would have a net production cost of about 26 rupees if the seed pods are bought at 6 rupees per kilogram and every scrap of seed and seed pod is converted into something valuable, with the seed going into oil, the bi-product seed cake into fertilizer and the seed husk into a high- density brick that can be burnt for fuel.</p><p>The wide governmental support has also attracted substantial business interest. D1 Oils, a UK-based biodiesel producer, is the world's largest commercial jatropha cultivator, responsible for around 81,000 hectares of jatropha in Chhattisgarh and in the southern state of Tamil Nadu, with plans for an additional 350,000 hectares over the next few years. “The entire programme revolves around the government-funded jatropha seeds,” says Sarju Singh, until recently managing director of D1 Oils India. “The government gives farmers free or subsidized seedlings and D1 Oils guarantees to purchase the seeds at the price prescribed by the state.” The company claims to have invested more than £3 million (US$6 million) in plant science and financing its share of the plantings, which are joint ventures. </p><p>Cautious approach</p><p>Jatropha is already under cultivation in Tamil Nadu, India, where it can be grown with other crops such as sunflowers.G. JAWORSKY</p><p>Source: United Nations Development Programme/World Bank. Jatropha figure from Indian Planning Commission Yet most of these plantings have yet to reach whatever maximum level of productivity they might eventually attain — the plants need a few years to bed in. And Ghosh is wary of subsidizing jatropha too much before mass cultivation of the plant is fully understood. “A lot of government funds may go down the tube,” he warns. Ghosh doesn't want the farmers to take on too much risk, so he is suggesting that they intersperse jatropha between their current crops, rather than banking on it as a cash crop. Shukla has similar reservations. “My immediate concern,” he says, “is that because the seeds are derived from wild plants there </p><p>5/53 0a3c5a2395ed61be11492a7dbf31b8fe.doc 2018/1/9 is no assurance of yield.” Shukla says the CBDA, like Ghosh, is promoting jatropha as something farmers limit themselves to planting between their rice fields. The only situation where all are agreed that it makes sense for small farmers to cultivate whole fields of jatropha is on farm land that has become or is becoming unproductive. It is a good fallow crop, says Becker: “It has a deep root system which stops ground erosion and increases water storage in the soil.” This, he says, leads in turn “to more biomass growth and an accumulation of organic carbon in the soil”.</p><p>Henk Joos, D1 Oils' director of plant science and agronomy, agrees that assured yields and the techniques needed to achieve them on a large scale need a lot more research. Yield estimates currently vary a great deal. India's Planning Commission estimates about 1,300 litres of oil per hectare, but Ghosh, conservatively, foresees a figure of about half that. Yield research is the main focus of D1 Oils' Indian operations, he says. The company is currently testing a number of jatropha varieties to see which ones grow best in India's varied climatic zones. “It will be two or three years before we get real scientific data to base an industry on,” he says. “We are not there yet, we have a lot of work to do.”</p><p>This is the sort of work Ghosh is currently overseeing at the CSMCRI's test plots. “It isn't the most glamorous work, but the mass multiplication of reliably producing plants is key to developing an industry, he says. Ghosh and his team are looking at precisely what kind of soil conditions and just how much water jatropha needs in order to reliably pump out oil-bearing seeds. The fact that jatropha plants can survive droughts does not mean they will not be more productive if they get more water. The optimum amount of water is still unknown.</p><p>STRINGER INDIA/REUTERS The team is also continuously on the lookout for plants that could be potential progenitors for a generation of a high-yield crop. “We have one plant which has given us 5 kilograms of seed,” says Ghosh. “We have yet to get that from any other plant.” The CSMCRI is trying to perfect the use of shoot-tip cuttings as a means for mass-replication of jatropha plants so it can capture their best attributes. Culturing tissue cuttings from the plant's growing tip, says Ghosh, is the most reliable means of propagating exact copies of a parent plant, an important step in creating an army of dependable high-yield clones. It's a common enough technique — but like so much technology, it hasn't yet been reliably adapted to jatropha. “The problem is, we just don't have the protocol right,” says Ghosh.</p><p>These various efforts are not part of any overarching plan. Despite the general enthusiasm for India's national mission on biofuel, there is a definite lack of cohesion at the national level. “Right now, ad-hoc research is being done by different agencies,” says Chopra, “but it doesn't add up, because they each do their own thing.” A national biofuel policy that was written by Chopra and his colleagues shortly before his retirement might help. It envisages an authority that would coordinate research and provide funding through various government agencies in order to cultivate jatropha on an industrial scale. But this policy, like </p><p>6/53 0a3c5a2395ed61be11492a7dbf31b8fe.doc 2018/1/9</p><p> the national mission on biofuel, has yet to go through the cabinet. In this case, it has been stalled by disagreements between various ministries on how to price jatropha — the Ministry of New and Renewable Energy suggests subsidizing seeds; other government ministries suggest subsidizing biodiesel itself. But, says Chopra, “I expect it will come together, perhaps this year or early next year”.</p><p>Ghosh remains cautious and optimistic in level-headedly equal measure. “We must neither get carried away by hype nor get despondent if the initial results of cultivation are not as per expectation,” he says. “The future will depend on how seriously and scientifically we pursue our goals.”</p><p>See Editorial, page 637. . Daemon Fairless is this year's winner of the IDRC- Nature fellowship.</p><p> References</p><p>1. Francis, G., Edinger, R. & Becker, K. Nat. Res. Forum 29, 12–24 (2005). | ISI | 2. Foidl, N., Foidl, G., Sanchez, M., Mittelbach, M. & Hackel, S. Bioresource Technol. 58, 77–82 (1996). | Article | ISI | ChemPort | 3. Ghosh, A. et al. Int. J. Environ. Stud. — special issue on India's future energy options (in the press). </p><p>Comments</p><p>Reader comments are usually moderated after posting. If you find something offensive or inappropriate, you can speed this process by clicking 'Report this comment' (or, if that doesn't work for you, email [email protected]). For more controversial topics, we reserve the right to moderate before comments are published.</p><p> Jatrophraud. Those hyping this species rarely give its common English names (vomit nut or purge nut) nor those of its oil (hell oil or oleum infernale). It has surface irritants, yet must be hand picked and dried and the nuts removed by hand from the outer coating. Its pollen is allergenic, it contains a protein curcin (similar to ricin- and as with castor beans, eating not too many seeds is lethal), and its oil has some pretty awful components. Most of the problems could be rectified transgenically. While there is no regulatory scrutiny of the dangerous wild type being cultivated, the regulatory costs to render it safer and easier to cultivate would be prohibitive. The rural poor will not be richer from growing an unmodified, undomesticated crop such as this, they will just be less healthy. Jonathan Gressel o Report this comment o 10 Oct, 2007 o Posted by: Jonathan Gressel </p><p>7/53 0a3c5a2395ed61be11492a7dbf31b8fe.doc 2018/1/9</p><p> Gressel you are the fraud. I wonder if perhaps you can speak of your years of field experience or the 71.38% reduction in carbon emissions from the jatrobased biodiesel BA100. Or the Millions of Rupees now going into the hands of farmers instead of OPEC's greedy little hands. I have seen this and so much more with my own eyes using non-GM crops in India and Indonesia. Yes, if you drink it you will get sick its feedstock for biofuel what do you expect. The road to solving global warming will be beset upon all sides with people that want to stand on there soapbox and pronounce it all a hoax. Without a shred of any real credability or experience. If anyone would like to see photographs, facts or figures from my research please e-mail me. [email protected] o Report this comment o 10 Oct, 2007 o Posted by: Tyson Bennett </p><p> The reader is invited to official and peer-reviewed sources to ascertain the toxicity of Jatropha. The oil was initially claimed to contain a fatty acid curcanoleic acid, structurally and functionally related to ricinoleic and crotonoleic acids, and like them, is a of skin tumors 1. The irritant/cancer potentiator/synergist seed oil is now known to contain 0.03 and 3.4% curcusones, irritant diterpenoid phorbol esters. The best extraction procedures available for the removal of the phorbol esters remove about half 2, which is unacceptable toxicologically in accessions with high initial content. As jatropha seeds have a pleasant taste, the plants are particularly attractive to children 1, possibly because the seeds contain dulcitol and sucrose 3. Numerous cases of toxicoses from the toxicalbumin lectin (curcin) are reported in the medical literature and ingesting four seeds can be toxic to a child, with symptoms resembling organophosphate insecticide intoxication, yet with no known antidote for the lethal mixture 1. Some selections have been performed to find accessions that are less poisonous. The results are still quite poisonous, probably because the screening was performed to assay amounts of a single poisonous component, forgetting that jatropha contains a suite of toxic compounds. For example, a “non-toxicâ€� Mexican variety has 5% the amount phorbol esters, but still has half the amount of toxic lectins as the toxic varieties, and about 25% more trypsin inhibitors and 50% more saponins 4. The poisons could all be removed using RNAi technology, and the meal would then be appropriate for animal feed, and not as a dangerous environmental pollutatnt. 1. INCHEM. Jatropha curcas L. Intl. Programme Chem. Safety http://www.inchem.org/documents/pims/plant/jcurc.htm (1994). 2. Haas, W. & Mittelbach, M. Detoxification experiments with the seed oil from Jatropha curcas L. Industrial Crops and Products 12, 111-118 (2000). 3. Gübitz, G. M., Mittelbach, M. & Trabi, M. Exploitation of the tropical oil seed plant Jatropha curcas L. Bioresource Technology 67, 73-82 (1999). 4. Makkar, H. P. S., Aderibigbe, A. O. & Becker, K. Comparative evaluation of non-toxic and toxic varieties of Jatropha curcas for chemical composition, digestibility, protein degradability and toxic factors. Food Chemistry 62, 207-215 (1998). o Report this comment o 11 Oct, 2007 o Posted by: Jonathan Gressel </p><p> Jatropha curcas: toxic disaster or fuel for the future? Bennett and Gressel both have a point. Although even some basic agronomic characteristics of J. curcas are not yet fully understood, the plant enjoys a booming interest, and this may hold the risk of unsustainable practice. While our qualitative sustainability assessment, focusing on environmental impacts and to a lesser extent on socio-economic issues, is quite favorable as long as only degraded land is taken into J. curcas cultivation, there are several tradeoffs between different sustainability dimensions cautioning us against jumping on the Jatropha Express too soon. Please see Achten WMJ, Mathijs E, Verchot L, Singh VP, Aerts R, Muys B (in press) Jatropha bio-diesel fueling sustainability? Biofuels, Bioproducts and Biorefining, at http://www3.interscience.wiley.com/cgi-bin/jissue/114204229</p><p>8/53 0a3c5a2395ed61be11492a7dbf31b8fe.doc 2018/1/9</p><p> o Report this comment o 11 Oct, 2007 o Posted by: Raf Aerts </p><p> Your article was very well researched and indeed offers to India (and other Countries with equal requirements for providing alternative sources of fuel to fossil derived fuels) a temporary respite from 'Peak Oil.' With the developments in India moving forward apace and the population likely to exceed that of China in less than twenty years and the aspiration of all to have personal transport there will surely be a need for more fuels including substitutes for refined fossil fuels Diesel and petroleum/gasoline. I wonder then though whether the authors and researchers have taken note of the other potential sources of Biofuels available to India. One of these is Pongamia - the Indian Beech Nut - which was recognised by Dr. Udipi Shrinivasa from the Indian Institute of Science, Bangalore as being of equal importance for its oil derived fuel to be a useful source of Bio-Diesel and an equal substitute for fossil fuel derived Diesel. From the reading of his work it would appear that this could be of more importance to the Indian economy than Jatropha. Yet in all of these issues about the developments of BioFuels little if ever mention is made of the fact that growing crops on land that might also be used for growing foods has its disadvantages. With the scarcity of such land across the world we should be careful in all that we do in case we upset the fine balance between ecosystems in the area. Perhaps the two events in recent time that should be referred to as a warning in this area are the destruction of the Aral Sea basin as a result of the diversion of the source rivers to feed the Cotton Industry and the effects of the Communal Farming initiatives in China during the 1950s and 1960s. (There will be others in historical terms of equal note!) In respect to the options for the other Biofuel substitute - Ethanol - for petrol/gasoline there is as much an obvious need in India as there is elsewhere in the World (be it China, Korea, Indonesia, Nigeria, Brazil and the rest of the South Americas or in the 'Western Nations.') The traditional route to manufacture Ethanol again relies on the use of crops that are primary sources of food (Sugar Cane and Beet or Corn, Wheat, Rice and Grains etc.) or they are grown on land that should preferentially be used to grow food. This conflict between the use of Crops (and land) for the Production of Fuel or Food will bedog us for some time unless we take action to redress it at the earliest opportunity. One of the easiest ways to do this is to intercept the Biomass from Waste sources and use this to manufacture the fuel Ethanol. The process to do this Mild Acid Hydrolysis is well founded and established having been developed in the late 19th Century and early 20th Centuries to make batch small quantities of Ethanol for transport in the USA and Europe prior to the development of the mass development of cheap oil in the USA and the European North Sea. With the developments of the process in recent time by Genesyst and the use of its Internationally patented Gravity Pressure Vessel the process is now continuous and the resulting efficiency of conversion of Biomass to Ethanol means that it can be used on any sources of Biomass including that found in Waste (previously considered unusable) in an emission free environmentally acceptable and economical way at around a quarter of the cost of the Thermal Destructive - or the Incineration - and Waste to Energy options. The sources of the raw material available to make Ethanol now includes Biomass found in Waste from Agriculture and Farming, Forestry, Food Production and Discards, Commerce and Industry (including Saw Mill and Paper Manufacture, etc.), and Construction Debris and the likes. Importantly though for Society it includes the Biomass we discard in our Municipal Solid Waste. This source of Biomass is available from every community around the World, and it is a constant source of material that is not affected by Climate, Seasons or Internationally defined Commodity Prices established outside the Country of production. In the Metropolitan City of Mumbai some figures were quoted by Surika Kamil (for the Indira Gandhi Institute of Development Research) which gave an insight into the issue for Greater Mumbai as follows. In 2001 the total daily production of Municipal Solid Waste was 6260 tonnes (rounded up to the nearest 10:) The Biomass equivalent in this same source of Waste was 3950 tonnes: Assumed Water Content of the Biomass as a percentage 50% Potential Ethanol yield at 200 litres per dry tonne of Biomass = 144+ million litres per year. If it was possible to consider all the potential Municipal Waste collected in the major cities in India then from the report given in the Hindu on Wednesday March 07th 2007 where it is stated '..."The present annual solid waste </p><p>9/53 0a3c5a2395ed61be11492a7dbf31b8fe.doc 2018/1/9</p><p> generated in Indian cities has increased from 48 million tones in 1997 to 95 million tonnes, which might exceed 150 million tonnes over the next seven years," says Mr. Dhoot.' Supposing just half of this 150 million tonnes was made into the fuel Ethanol then the comparative quantity of Ethanol derived would be nearer 4700+ million litres of Ethanol (a not insignificant sum)! And by adding to this other waste sources of Biomass it is possible that even India could become self sufficient in substitute Biofuels. The comparison elsewhere around the world though is even more startling. City/Population/MSW tonnes per day/Ethanol litres per year Buenos Aries/12.4m/7,800/480,000,000 Beijing/15.3m/13,300/820,000,000 São Paulo City/18.7m/15,900/985,000,000 MetroSeoul/23.9m/22,800/1450,000,000 If Brazil was to exploit the production of Ethanol from Municipal Waste it could increase the total country's production very significantly! By converting Biomass from its Municipal Waste to Ethanol Metro Seoul could replace over 40% of the total petrol used in the area. So in returning to the article in the current press We should be looking at the wider picture when it comes to addressing the potential of Biofuel production. This should embrace the use of Biomass as it does not impinge on the use of Crops that should primarily be used for Food or grown on land that ought in the first instance be used for growing food. The use of Jatropha (or indeed Pongamia) may be such a crop but even then in certain areas it may have distinct advantages for Society. o Report this comment o 11 Oct, 2007 o Posted by: Peter Hurrell </p><p> Thanks for the nice, well researched reviews on Jatropha. Biodiesel from Jatropha oil is a huge potential to reduce the spread of desert and cover arid/semi-arid land with green shrubs. It also can enable poor, rural people in countries like India, Chain, Africa etc to get some extra earnings. Though I have enough doubt if it can solve the problems regarding fossil fuel in future in large scale, even in those countries. The main bottleneck for using Jatropha is very high labour requirement collect seeds. This will be real long- term problem for its use in industrialized countries where labour cost is very high. Seed cakes of jatropha is known to contain toxicity and not suitable for cattle feed and fertilizer. In countries like India, jatropha has invited private investment. Their goals are not necessarily the elevation of rural poverty and provide green cover for arid and semiarid land. They are mostly interested to gain from Govt subsidy associated with jatropha plantation. This also has the potentiality to force and exploit poor and mostly illiterate farmers in villages to cultivate jatropha instead of their normal crops. This will be of great concern in countries like India with huge population to feed. Unless proper and systemic studies are completed to ascertain its agronomic and environmental impact, economic feasibility (mainly for rural mass), Jatropha should not be allowed for mass cultivation. Its use by private investors also should be properly monitored. o Report this comment o 11 Oct, 2007 o Posted by: jayanta chatterjee </p><p> I am really interested to see these opinions about Jatropha curcas, not least because I am involved in an EU AidCo funded project called RE-Impact (www.ceg.ncl.ac.uk/reimpact) in which we are planning to develop integrated tools and methodologies to help stakeholders and policymakers see the bigger picture when planning energy plantations. We are looking at water resource, socioeconomic, biodiversity and climate change (CDM and JI) impacts from global to local scales, with case studies in India, China, Uganda and South Africa. As discussed in the article Jatropha is already planted up in a big way in India and China with more planned, whilst interest in Uganda is scant and there is currently a moratorium on the crop in South Africa. All very interesting stuff, and we hope to develop the project meaningfully over the next 3 years with input from any interested stakeholders at any level. For more information, workshop details, or to get in touch, please do go to the website.</p><p>10/53 0a3c5a2395ed61be11492a7dbf31b8fe.doc 2018/1/9</p><p> o Report this comment </p><p> o 12 Oct, 2007 </p><p> o Posted by: Jennifer Harrison </p><p> Biodiesel, like starch-based ethanol, uses only a fraction of the plant. A winning strategy for biofuels would be based on converting the cellulose.</p><p> o Report this comment </p><p> o 12 Oct, 2007 </p><p> o Posted by: Paul Braterman </p><p> The perspective on Jatropha I mentioned above, has been published online and is available via http://dx.doi.org/10.1002/bbb.39</p><p> o Report this comment </p><p> o 20 Nov, 2007 </p><p> o Posted by: Raf Aerts Add your own comment</p><p>You can be as critical or controversial as you like, but please don't get personal or offensive, and do keep it brief. Remember this is for feedback and discussion - not for publishing papers, press releases or advertisements, for example. If you ramble on in an annoying way too often, we may remove your posting privileges.</p><p>Although you are an existing nature.com user, you will need to agree to our Community Terms and Conditions before you can leave a comment.</p><p> View and accept terms and conditions </p><p>Published online 20 February 2008 | Nature 451, 880-883 (2008) | doi:10.1038/451880a </p><p>News Feature Energy: Not your father's biofuels</p><p>If biofuels are to help the fight against climate change, they have to be made from more appropriate materials and in better ways. Jeff Tollefson asks what innovation can do to improve the outlook.</p><p>Jeff Tollefson </p><p>11/53 0a3c5a2395ed61be11492a7dbf31b8fe.doc 2018/1/9</p><p>D. SIMONDS Biotechnology has changed the way that drugs are discovered, designed and, often, made. It has spread new capabilities across the farms of much of the world, sometimes amid much controversy. Now some of its advocates are suggesting that it is poised to overhaul the energy sector as well, changing both the crops that are grown and the fuels that are made from them.</p><p>Entrepreneurs have attracted hundreds of millions of dollars for bio-energy companies working on 'second-generation' fuels produced from crop residues, grasses or woody materials that avoid the shortcomings of ethanol distilled from corn starch or biodiesel produced from oil crops. Some are offering hope for higher yields from less land, from more marginal land, and with less investment in terms of energy and fertilizer; others are promising fuels better suited to the needs of drivers and the existing fuel infrastructure. Even the major oil companies are getting involved.</p><p>“The market is slated to be so big that there will be opportunities for multiple approaches, and it will probably take many years before we settle on one absolute best approach,” says Doug Cameron, chief science officer for Khosla Ventures, a venture-capital firm in Menlo Park, California, that is backing a large number of start-up bioenergy companies.</p><p>Liquid biofuels will never make up a significant portion of the global transportation fuel supply unless biologists and engineers make the most of these opportunities. During the past four years alone, global ethanol production has more than doubled to nearly 50 billion litres (about 13.2 billion gallons) in 2007. Biodiesel, although starting out much lower, nearly quintupled to 9 billion litres (2.4 billion gallons) during the same period (see A growing concern). This rate of growth is not sustainable — and with current production methods far from desirable, because the agricultural techniques used often damage the environment on a scale that far outweighs any good achieved through the biofuels' use.</p><p>In the United States, which has ramped up production and is now home to the world's largest biofuel industry, roughly 23% of the corn crop goes to ethanol, which in turn provides 3% of the nation's transportation fuels, according to Alex Farrell, an energy and resource scientist at the University of California, Berkeley. Worldwide, biofuels make up less than 1% of transportation fuel, he says. Current technologies could push that as high as 2–3%, “but anything much larger than that will have to be based on significantly different technologies”. </p><p>Fuels for the future</p><p>Applying biotechnology to biofuels is not a new idea. In 1991 Lonnie Ingram, a microbiologist at the University of Florida, Gainesville, was awarded a patent for an engineered Escherichia coli bacterium that converts sugars into ethanol. But only now is the technology really coming into its own — not least because today's 'metabolic engineering' and 'synthetic biology' put much more ambitious fuels than ethanol on the table, or indeed into the pipeline.</p><p>12/53 0a3c5a2395ed61be11492a7dbf31b8fe.doc 2018/1/9</p><p>“I've always been of the opinion that ethanol is for drinking, not driving,” says Jay Keasling, a chemical engineering professor at the University of California, Berkeley, who has pioneered the synthetic biology needed to get microbes to produce various new classes of molecule. To take one example: drinking is made easy by the fact that ethanol and water mix easily. That's good for scotch and soda, but bad for pipelines, which give the fuel a chance to get watered down and contaminated. In the United States, home to the largest biofuels industry in the world, trucks or train cars carry the fuel from the agricultural heartland where corn is grown to the coastal areas where fuel is needed most. In Brazil, the other biofuel giant, where sugar-cane ethanol is produced much more efficiently, the agricultural source and the urban users are relatively close together in the country's southeast — one of the many advantages the Brazilian industry enjoys.</p><p>This is why Amyris Biotechnologies, a start-up company in Emeryville, California, of which Keasling was a co-founder, and a number of other small companies in California and Massachusetts are designing microbes to make better fuels — fuels with higher energy contents that are better suited to pipelines and other infrastucture. University researchers are also in the race. Last month Jim Liao, a researcher at the University of California, Los Angeles, described an E. coli in which more than a dozen modifications to the metabolic pathways normally used to produce amino acids caused the bacteria to produce isobutanol, an alcohol with four carbon atoms to ethanol's two, and similar molecules. Even without optimizing every step of the process, Liao's lab was able to achieve a yield of 86% of the theoretical maximum (S. Atsumi, T. Hanai & J. C. Liao Nature 451, 86–89; 2008).</p><p>His process has been licensed by Gevo in Pasadena, California, a start-up company funded by Khosla Ventures, among others, which says that it hopes to begin commercial-scale production within a few years. Gevo chief executive Pat Gruber says that the technology could be retrofitted on an existing bioethanol plant for as little as US$20 million: “We're not screwing around — the basic science is done. We are going to try and get this stuff developed and into the marketplace.”</p><p>Another form of butanol has attracted oil-giant BP and DuPont, one of the world's largest chemical companies, which are working together on biofuels. Butanol has a higher energy density than ethanol, offering roughly 85% of the energy content of a standard petrol mix, compared with about 66% for ethanol; this offsets the fact that less butanol than ethanol can be made from a given amount of biomass (there are only so many carbon atoms to go round). Although other molecules could pack even more energy, DuPont officials say that they settled on butanol as a molecule that meets their needs and can be developed quickly.</p><p>DuPont has a track record in the sort of metabolic re-engineering required for such things: the E. coli that churn out propanediol, a chemical used in various materials and industrial processes, in its facility in Loudon, Tennessee, have had 30 changes made to their metabolic pathways. It took about 11 years for that project to get from the proof of concept to production, but officials say biobutanol could be sorted out much faster. “In the old days, it would take four to six months to clone a new gene,” says John Pierce, DuPont's vice-president for applied biosciences. “Now it takes two weeks and you usually do it by mail.”</p><p>13/53 0a3c5a2395ed61be11492a7dbf31b8fe.doc 2018/1/9</p><p>View an enlarged version of the graph.</p><p>Butanol's advocates say that their fuel could be run through a refinery to produce longer chain molecules as desired. Amyris and LS9 of Cambridge, Massachusetts, are looking to skip this step and move straight to molecules more like those that engines already burn. Both companies specialize in synthetic biology — which aims to create new biological entities in a much more thoroughly designed way than traditional genetic engineering — and both have received backing from Khosla Ventures, among others.</p><p>LS9 was co-founded by George Church, a geneticist at Harvard Medical School in Boston, Massachusetts, and Chris Somerville, a plant biologist at Stanford University in California, who previously headed the Carnegie Institution's department of Plant Biology. Somerville is currently heading the new Energy Biosciences Institute (EBI), a partnership between the University of California, Berkeley, Lawrence Berkeley National Laboratory and the University of Illinois at Urbana-Champaign. The EBI was kick-started in 2006 with a $500 million pledge from BP. </p><p>Different pathways</p><p>LS9 wants to transform the fatty acids naturally produced by E. coli into specific hydrocarbon fuels; it has announced plans for a pilot plant and says that commercial production could begin within two to three years. Amyris decided to take yeast, which is known for its ability to make ethanol, and train it to produce longer-chain molecules such as gasoline, diesel and jet fuel.</p><p>The company has engineered roughly 1,000 strains of yeast so far and expects to build as many as 2,000 more in the months ahead. When a strain looks promising, the scale-up begins, as molecular biologists hand their product to chemical engineers and fermentation engineers. In addition to producing exactly what is needed, Jack Newman, Amyris's senior vice-president for research, says that the technology could prove to be extremely versatile. “You could have your plant making diesel fuel one day and almost literally turn around and make jet fuel the next.” </p><p>Wood pulp and fiction</p><p>All these companies are looking at traditional raw materials for their wonderbugs — sugars. And this means that although they may make more attractive and easily distributed fuels, they will not of themselves change the industry's </p><p>14/53 0a3c5a2395ed61be11492a7dbf31b8fe.doc 2018/1/9 productivity. To do that requires cheaper and more sustainable feedstocks than sugars and starches from cane and corn.</p><p>“I'm of the opinion that the crucial thing that needs to be done is not actually to make better fuels out of sugars but to make sugars more efficiently from cellulose,” says Lee Lynd, a biology and engineering professor at Dartmouth College in Hanover, New Hampshire. “The thing that limits corn ethanol, frankly, is not ethanol — it's corn. Those other molecules will become important in the broader scheme of things if and when we solve the problems with cellulosic biomass.”</p><p>Turning cellulose — the tough polymer from which the cell walls of plants are made — into biofuel is currently a major focus in the industry. It widens the possible range of feedstocks greatly, making it possible to use crops for biofuels that are not also food for humans. Legislators are keen to push the industry in that direction. The ethanol mandate enacted by the US Congress in 2007 requires that, of an annual ethanol production of 136 billion litres (36 billion gallons) required by 2022 — more than five times current US production — 44% must come from cellulosic foodstocks, with corn's contribution remaining static from the mid 2010s. Things might be able to go even further. A report by McKinsey & Company, a consultancy firm based in New York, suggests that, at oil prices above $70 per barrel, cellulosic feedstocks could supply half of the global transportation fuels by 2020 (although that figure explicitly ignores many real- world constraints).</p><p>The fly in this ointment is that the world cannot yet boast a single commercial- scale cellulosic-ethanol facility. Breaking cellulose down into sugars is not easy work, and can use up a lot of energy; what's more, not all the sugars produced are easily fermented. Despite the recent spike in oil prices on the international market, lenders and investors have hesitated to pump money into commercial- scale ventures, fearing technical risks and a potential drop in the price of oil. To help push the technology over the first economic hurdle, the US Department of Energy (DOE) is pumping $385 million into six demonstration projects. Work begun on the first of them, run by Colorado-based Range Fuels, in Georgia last autumn.</p><p>15/53 0a3c5a2395ed61be11492a7dbf31b8fe.doc 2018/1/9</p><p>R. KALTSCHMIDT/LBNLE Cellulose can be broken down with heat and catalysts. It can also be broken down with biology. This is where biotechnologies can come in. Dartmouth's Lynd is the co-founder and chief scientific officer for Mascoma Corporation in Cambridge, Massachusetts, which is pursuing work on a bacterium that can produce the enzymes to break down cellulose on demand as part of the process. Verenium Corporation, also in Cambridge, the first publicly held cellulosic-ethanol enterprise, is developing both new enzymes and organisms to break down the structural tissue. Other companies and researchers are looking to the microbes that break down cellulose in the guts of cows and termites, or to fungi — that is to say, the natural world's most accomplished consumers of the cellulose found in grasses and wood. </p><p>Finding a feedstock</p><p>If cellulosic technologies can be made to work, there's still the question of where the cellulose comes from. For the past ten thousand years, most human meddling with the proclivities of plants has been designed to make them better to eat — more sugar in the cane, more starch in the corn, more protein. Energy crops, though, require a different approach. No need for fruit or seed — just for fast growing cellulose, ideally in a form that requires little or nothing by way of extra inputs such as fertilizer. Cellulose that is particularly easy to break down gets bonus points.</p><p>Switchgrass, a hearty, fast-growing grass native to America's prairies, is one much-touted possibility, but even a brief survey indicates that there could be better options. Steve Long, a professor at the University of Illinois at Urbana- Champaign, has spent years studying Miscanthus giganteus, a relative of sugar cane that is native to East Asia. He has studied the grass in Denmark, England and now Illinois, and says that the average yield is double that of switchgrass and 50% higher than corn — without any fertilizers.</p><p>16/53 0a3c5a2395ed61be11492a7dbf31b8fe.doc 2018/1/9</p><p>According to Long, meeting the ethanol goals laid out in 2006 by President Bush (which were a touch lower than the mandates actually passed by congress) with corn ethanol would require 25% of the nation's cropland. That figure drops to 15% if cellulosic technology allows you to make use of the corn 'stover' — the bits left over after harvest. It drops to 8% if you use M. giganteus — and such grasses could be grown on marginal land that is not being used for food production today.</p><p>And, according to Long, neither stover nor M. giganteus are the last words. “A lot of effort is being invested in modifying corn stover to make it easier for digestion, but I think we need to think much more broadly,” he says. “It seems very unlikely that we've come across the very best option.”</p><p>Whichever crops look most promising, engineers will be eager to tinker with them. Keasling says that a decade of intensive biotechnology could now do for an energy crop as much as centuries of selective breeding have done for many food crops. New models of cultivation may help too — for example the replacement of monocultures, which are useful if you want a particular form of food, with polycultures that simply maximize biomass. David Tilman and his colleagues, at the University of Minnesota in St Paul, reported last year that plots with a diverse mixture of prairie grasses yielded on average 238% more energy than plots with a single crop (D. Tilman, J. Hill & C. Lehman Science 314, 1598–1600; 2006). All these approaches might be tailored to marginal lands where the soil wouldn't support food crops.</p><p>Or you could just do away with soil altogether: that's the appeal of algae. GreenFuel Technologies, based in Cambridge, Massachusetts, is developing algal bioreactors that tap into carbon-dioxide streams from coal plants to produce rich algal crops that can be harvested and turned into biofuels. A more ambitious approach would be to have the algae act as both feedstock and processor, secreting ready-made fuels. Tasios Melis at the University of California, Berkeley, is looking at getting algae to secrete hydrocarbons in a form that can be continuously collected.</p><p>As it is today: this VeraSun Energy plant near Aurora, South Dakota, produces 450 million litres of corn ethanol a year.E. LANDWEHR/AP Botryococcus braunii, the green alga that Melis is working with, naturally secretes a 30-carbon terpenoid that can be processed into fuel, perhaps in order to reduce the effects of ultraviolet light. Melis says that he has devised a method for collecting the product — a sticky film to which the algae adhere — and is now working to increase production. More ambitiously, various groups, including Melis, are looking at having algae produce hydrogen.</p><p>Another approach, taken by Coskata in Warrenville, Illinois, (yet another Khosla Ventures start-up) and its partner General Motors, is to avoid putting effort into specialist feedstocks and instead develop a process that works universally. Coskata's process converts carbon-based feedstocks, which could be crops, agricultural waste or municipal waste, into carbon monoxide and hydrogen. This is the first step of processes by which coal or natural gas are turned into liquid fuels, a procedure that is normally taken as an alternative to biological means for </p><p>17/53 0a3c5a2395ed61be11492a7dbf31b8fe.doc 2018/1/9 producing fuels. Coskata says it has a way of combining the two approaches, with microbes that turn the 'synthesis gas' straight into ethanol. The company is currently developing a pilot project that it says will be able to produce ethanol for less than 26 cents per litre ($1 per gallon). </p><p>Scaling up</p><p>One thing all these innovations share is that they have yet to be attempted on a large scale. Biotech may work well for drug companies that sell small volumes at premium prices, but the economics of biofuels are more in line with the oil and gas industries, which sell in bulk for low prices and are dominated by the cost of raw materials and manufacturing.</p><p>ADVERTISEMENT</p><p>One of the problems is that microorganisms evolved to take care of themselves, not people. Re-engineering their metabolisms in such a way that they excrete fuels — which by definition are energy-rich compounds — means convincing them to forgo energy that they might otherwise use to their own ends. Moreover, in many cases the fuels can be toxic to the organisms themselves. All this provides ways for the engineering to come unstuck.</p><p>“If [the microbes] are unhappy with what they are doing, they are going to evolve away from what you want them to do,” says genome entrepreneur Craig Venter, whose company Synthetic Genomics in La Jolla, California, has an interest in biofuel production. “A key part of the future is going to be designing a system where they are not grossly unhappy with what they are doing.” Many researchers see hope in producing longer-chain biofuels precisely because it decreases the stress on the microbes doing the work. Ethanol is poisonous to its producers (which is why fermentation can't on its own produce hard liquor — the yeast dies). Longer-chain molecules will separate out from the medium in which their producers grow; this avoids the costly step of distillation.</p><p>Even if the microbial producers can be kept happy, there's a lot more work to do in producing systems that will work on an industrial scale in commercial refineries. “Among scientists it's considered very doable,” says Michael Himmel, who heads a team of researchers working on cellulosic ethanol at the DOE's National Renewable Energy Laboratory in Golden, Colorado. “It's difficult work and it's going to take some funding. But it's not cold fusion.” But what's doable in principle to a scientist is not always practical to an engineer.</p><p>18/53 0a3c5a2395ed61be11492a7dbf31b8fe.doc 2018/1/9</p><p>Big oil companies, which would have the know-how for such engineering, are keeping an eye on the field. As well as BP's work with DuPont, Shell is partnering with Iogen in Ottawa, Canada, which ran one of the DOE's cellulosic pilot plants, and with an algal biofuel producer in Hawaii. But all this is small compared to the investments such companies make in their oil-based business.</p><p>D. SIMONDS Biofuels will never take over the whole liquid-fuel market, let alone amount to a large proportion of total energy use. But they, and other technologies, have a part to play. A decade and a half after receiving his patent on an ethanol- producing bacterium, Ingram is still a biofuel enthusiast. But he's also a realist. In the same law that expanded the ethanol mandate, Congress also increased the fuel-efficiency requirements for vehicles by 40%, pushing the average efficiency required by 2020 up to 6.7 litres per hundred kilometres (35 miles per gallon) from the present average of 9.4 litres per hundred kilometres (25 miles per gallon). The technologies to do that are already available — Japan had an average fuel efficiency of 5.1 litres per hundred kilometres in 2002. And as Ingram points out, “If we increase gas mileage by 1 mile per gallon, that is about equal to all the ethanol we are making right now from corn.”</p><p>Jeff Tollefson covers climate, energy and the environment for Nature. See Editorial, page 865. </p><p>Comments</p><p>Reader comments are usually moderated after posting. If you find something offensive or inappropriate, you can speed this process by clicking 'Report this comment' (or, if that doesn't work for you, email [email protected]). For more controversial topics, we reserve the right to moderate before comments are published.</p><p> I was surprised by the lack of mention of Searchinger and Tilman's recent Science papers on the enormous breakeven times of biofuels. We unfortunately don't have decades to solve our greenhouse gas emissions problem. Biofuels will also have a tough time supplying anything but a small fraction of our energy because plants are so inefficient. Fundamentals of Renewable Energy Processes gives the solar energy to ethanol conversion efficiency of sugarcane (one of the best) as 0.13%. If you compare this to the 30% of a Stirling dish, you've got a factor of 231. Worse, ethanol, butanol, etc. are converted into mechanical energy far less efficiently (much less than half) than the output from a Stirling dish (heat engines are subject to the Carnot limit after all). All of this translates into enormous differences in land area to create the same amount of work. In my opinion, the land area requires should be a primary criteria for evaluating renewable fuels. o Report this comment </p><p>19/53 0a3c5a2395ed61be11492a7dbf31b8fe.doc 2018/1/9</p><p> o 20 Feb, 2008 o Posted by: Earl Killian </p><p> There is talk in this article of growing sources of cellulose on “marginal landâ€� or with no fertilizer inputs. However, unless you recycle the wastes from the energy production process back to the soil the cellulose was grown in it will eventually become depleted for e.g. phosphate – and growth of plants that even tolerate low phosphate levels will become restricted. This is not a small problem since a Hubbert linearization analysis of mineral phosphate extraction predicts that this resource is 75% depleted! ( See www.energybulletin.net/33164.html and www.energybulletin.net/40300.html ) Phosphate extraction from lower quality sources would require greater energy inputs. This means that, if any phosphate for fertilizer is available in 10 years time, it will be exceedingly expensive. The looming phosphate shortage has enormous implications for biofuels and, more importantly for the continuation of western industrial agriculture. o Report this comment o 20 Feb, 2008 o Posted by: Michael Lardelli </p><p> The article failed to discuss that there is one cellulosic feedstock that is readily available and at present has negative environmental value, the 2 billion tons of straw available world wide. Straw eventually becomes carbon dioxide without providing value, and at the same time temporarily binds nutrients, causing farmers to overfertilize. Straw also harbors pathogens requiring fungicide use which is why it used to be burnt in the field. The use of this byproduct would not necessitate putting new land in cultivation. The major problem with all the crops being used as cellulosic feedstocks is that they have not been domesticated for use as biofuel feedstocks. The main problem is lignin, which prevents metabolism of cellulosics by the wonderful microorganisms being developed (as described by the author). All the cellulosics described must be pretreated with heat and acid before the microbes can be used. Reducing and modifying lignin by genetic engineering of the cellulosic crops, whether switchgrass, Miscanthus, or straw from crops, can substantially reduce the amount of pre-treatment, as has already been demonstrated in the pulp and paper industry in transgenically modified trees. The potential uses of biotechnology for developing biofuel feedstocks is described in a recent review: “Transgenics are imperative for biofuel crops. Plant Science 174: 246-263 (2008).â€� o Report this comment o 23 Feb, 2008 o Posted by: Jonathan Gressel </p><p> What do people think of this technology? They claim to have discovered a way to produce ethanol from more woody plants without requiring additionl energy. http://www.missionbiofuels.com/uploads/Announcement-Ligno-cellulosic%20Ethanol- 31108(final).pdf o Report this comment o 04 Nov, 2008 o Posted by: Kim Sweeny </p><p> Befouls are produced by anaerobic digestion of feed stocks that contains very small amounts of sulfur compounds. For example garlic and onions are very rich in sulfur. This produces very small amounts of highly toxic , flammable hydrogen sulfide , the worst green house gas that humanity does not need. Even sulfate ions in water used in the digestion process can produce this green house gas. It is millions time worst green house</p><p>20/53 0a3c5a2395ed61be11492a7dbf31b8fe.doc 2018/1/9</p><p> gas than CO2 or Methane. Hydrogen sulfide might be the culprit for the disappearance of the dinosaurs. A very simple example is when you eat garlic and onions you produce hydrogen sulfide that smells like rotten egg. This can explain the word holy cow. They had a camp fire during Christmas times after the loose cow consumed large amounts of onions. When the cow farted next to the camp fire , the blaze from the anus of the cow was very strong with yellow bluish color ( color of hydrogen gas with sulfur impurities). They called her holy cow. Mike Reda Consultant Saskatoon SK Canada</p><p>Published online 26 March 2008 | Nature 452, 400-402 (2008) | doi:10.1038/452400a </p><p>News Feature Chemistry: The photon trap</p><p>Chemists have long wanted to recreate photosynthesis in the lab — and to improve on its efficiency at converting sunlight into fuel. Katharine Sanderson reports on their latest efforts.</p><p>Katharine Sanderson </p><p>Fuel from the Sun: these silicon nanorods are designed to capture energy from sunlight to split water.B. KAYES, M. FILLER Solar cells can take sunlight and produce a current, giving instant power. But as soon as the Sun goes down, the lights go dim. If you could turn sunlight into fuel — to use for transportation or simply to store for later — you'd be on to a good thing.</p><p>Nature can already do this thanks to photosynthesis. Green plants take water, sunlight and carbon dioxide to make sugars and starches. This provides all the fuel they need, and most of the fuel we need too, in the form of food or oil.</p><p>The problem is that plants aren't very efficient at fuel making — only about 3% of the Sun's energy ends up as a useable fuel. And the fuel that works for plants doesn't necessarily work for us — the sugars and starches have to be further processed if our needs are more sophisticated than simply eating or burning.</p><p>But where plants excel is in getting electrons out of water to produce a fuel. A photovoltaic system, or solar cell, is simply a means of shifting electrons from one place to another. To make a fuel, the electrons are siphoned off, and stored in chemical bonds.</p><p>Plants get their electron supply from water. Chemists worldwide are trying to design synthetic systems that do the same. And the design they have to beat, or at least mimic, not only works at room temperature, it does so without the need for expensive metal catalysts. Making something cheap and similar to the machinery used by plants, the photosystem-II protein complex (PSII), remains a fundamental challenge.</p><p>21/53 0a3c5a2395ed61be11492a7dbf31b8fe.doc 2018/1/9</p><p>Some US chemists taking on the challenge are part of a collaborative effort called Powering the Planet, backed by the National Science Foundation. Three basic chemistry problems, each tackled by a different research team, form the crux of the project. One is to design an affordable material to collect energy from the Sun and convert it into current (led by Nate Lewis at the California Institute of Technology, or Caltech). Another is to perfect a catalyst at one end of the material to split water and produce oxygen (led by Dan Nocera at the Massachusetts Institute of Technology). And, the third is to design another catalyst at the other end to produce hydrogen, to be used as a fuel (led by Harry Gray, also at Caltech).</p><p>“We've made dramatic advances,” says Gray, who can be seen as the father of the project, having supervised both Nocera and Lewis as students in the 1980s. “We're not close to assembling the full device yet.”</p><p>To start the fuel-making process, sunlight hits a photo-active material. In plants this is chlorophyll, but in the lab it can be a silicon semiconductor, which has its electrons whacked out of position by the incoming photons. The dislodged electrons start to flow in one direction, creating a current. Left behind are positive charges, known as holes, and they drift in the opposite direction. This is a basic solar cell, which requires silicon of high purity, otherwise material defects cause the electrons and holes to recombine, reducing its performance. </p><p>Plant power</p><p>In the Powering the Planet design1, catalysts at either end of the semiconductor are used to drag the electrons and holes out of the system, preventing them from recombining with each other (see graphic). And water is added to provide the raw material for making the fuel.</p><p>One catalyst uses the holes in the semiconductor to drag further electrons from water. This process splits water, releasing oxygen and positively charged hydrogen ions (protons). These protons flow to the other catalyst, which combines them with the electrons in the semiconductor ultimately to make hydrogen molecules.</p><p>As well as providing hydrogen fuel for combustion, both the hydrogen and oxygen gases can be fed into a fuel cell — a means of reacting hydrogen with oxygen to produce water and electricity for powering an electric vehicle.</p><p>Harry Gray uses sunlight to make hydrogen fuel.B. LAI</p><p>22/53 0a3c5a2395ed61be11492a7dbf31b8fe.doc 2018/1/9</p><p>There are other ways to split water artificially. In 1975, Nobel prizewinning physicist Jack Kilby invented an electrolysis system that used power from a solar cell to drive electric current through a water-based solution (an electrolyte). This process produced protons and hydroxide ions, which reacted at the electrodes to make hydrogen and oxygen.</p><p>Aside from Kilby's simple system, more complex electrolysis cells have been built that use a photoactive semiconductor coated on one side with a platinum catalyst as one of the electrodes. When immersed in water, the semiconductor can both harvest light and generate the electrons and holes needed to split water into protons and oxygen. Hydrogen is released directly from the surface of the semiconductor, and oxygen is produced at a second platinum electrode.</p><p>This cell was built in 1998 by John Turner from the National Renewable Energy Laboratory in Golden, Colorado. His device converted water to hydrogen with 12.4% efficiency, four times as good as photosynthesis. But Turner had to use expensive materials such as platinum, the system had a lifetime of just 20 hours, and the hydrogen produced cost US$13 per kilogram2. “We can do better,” says Turner.</p><p>The problem with all electrolysis systems is that the electrode materials degrade rapidly in solution, and need to be replaced, increasing costs and decreasing efficiency. The main difference between Turner's cell and future technologies will be the materials used, with the precious-metal catalyst and expensive single- crystal silicon superseded by cheaper materials. “If we're going to solve this problem we can't use materials that are toxic or expensive,” says Gray. “This rules out most standard catalysts.”</p><p>Nate Lewis is leading Powering the Planet's light-harvesting effort. His team is refining a silicon material that he describes as “cheap and scaleable”. Instead of expensive single-crystal silicon, Lewis's photoelectric material is a carpet of nanoscale silicon rods, all pointing upwards. He's done this work with Harry Atwater, director of the Caltech Center for Sustainable Energy Research.</p><p>The rods are each single crystals, but the method used to grow them is much simpler than the precision wafer-processing technology needed for conventional solar cells. Atwater claims that this makes the rod silicon only as expensive as the silicon feedstock, at between $40 and $70 per kilogram.</p><p>The nanorods are also amazingly defect-free. “Once a nanowire begins to grow a little taller than it is wide, it expels defects,” says Atwater. This means that the only place where the holes and electrons could recombine is at the tip of each tiny rod.</p><p>In the device imagined by the Powering the Planet team, Lewis and Atwater's silicon-rod carpet will be held inside a plastic membrane. The catalysts are coated on opposite sides of the membrane to prevent the oxygen and hydrogen reuniting, potentially explosively. </p><p>Catalysing success</p><p>Harry Gray is making good progress at the hydrogen-producing end of the system. His catalyst is a cobalt molecule. “It works really well, with quite reasonable efficiency,” he says. This rather depends on your expectations. In catalysis, turnover rates are used to measure how many substrate molecules are converted to a product each second. Hydrogenase enzymes, which power the same reaction in plants, have turnovers of about 6,000 per second2, but Gray's </p><p>23/53 0a3c5a2395ed61be11492a7dbf31b8fe.doc 2018/1/9 catalyst is still a factor of a thousand less efficient than the hydrogenase enzyme, he says. “We have a proof of principle, but we have a long way to go,” says Gray.</p><p>Dan Nocera, at the Massachusetts Institute of Technology, Cambridge, is developing the oxygen-producing catalyst, which is proving to be the hardest part of the challenge. Nocera's team began by looking at expensive metals that are related to cheaper metals: a trick often used by chemists. Choose a target metal that could be potentially used, then look at its position in the periodic table and move down a row to a heavier, more expensive metal, where processes happen more slowly, and are more easily studied. Nocera has been using ruthenium, directly below iron. He hopes to transfer what he has learned to iron, copper or nickel, and is confident that this leap will happen soon, making a working system possible within five years, he says.</p><p>James Durrant, a chemist at Imperial College London, is also investigating water- splitting materials. He is well aware of the chemistry problems faced by Nocera in his quest for a cheaper catalyst. “Oxidizing water is vicious chemistry,” he says. The catalytic reactions involve molecules undergoing multi-electron processes, which are poorly understood. “As bad as it is to transfer one electron, the molecule is even more reluctant to give up the second electron,” explains Atwater.</p><p>This is because adding solar energy to water, and tying it up in molecules with higher energy bonds (oxygen and hydrogen) is what's known as a thermodynamically uphill process. And most of that uphill struggle happens at the oxygen-producing site. Making a single molecule of oxygen involves splitting two water molecules, and the whole process involves four electrons and four protons. “That's a lot of electrons and protons,” says Nocera. For this reason, Nocera says he doesn't want to simply copy photosynthesis. “It took two billion years of evolution,” he says. “I don't think I can do it in 20.”</p><p>Chemists want to replicate photosystem II (modelled above), used by plants to convert light into fuel.J. NIELD Other chemists are still trying to beat nature at its own game. A dozen European research partners, coordinated by Stenbjörn Styring from Uppsala University, Sweden, form the Solar-H network, funded by the European Union. They are looking closely at natural photosynthesis for inspiration.</p><p>To harvest energy from sunlight, the Solar-H team uses a ruthenium-centred molecule that helpfully absorbs light at a similar wavelength to chlorophyll. For the hardest part of the problem — the oxygen catalyst — the Solar-H team turns to the heart of PSII, which contains a molecule with four manganese atoms known as the oxygen-evolving complex.</p><p>Styring has been working on this problem for 15 years, and he has shown that it isn't necessary to fully replicate the oxygen-evolving complex. Instead, he thinks </p><p>24/53 0a3c5a2395ed61be11492a7dbf31b8fe.doc 2018/1/9 that just two manganese atoms are sufficient. Styring says he has recently had a breakthrough: a molecule that can split water into oxygen and protons, although the system is powered electrochemically, not by light.</p><p>Even after this prolonged effort, Styring expects criticism when he publishes the work, partly because the oxygen-evolving molecule isn't fully catalytic — the molecule is changed by the reaction and so probably cannot be reused. “People will be very sceptical, and so are we,” he says. “This is a very difficult field.”</p><p>He will have a hard time convincing researchers such as Durrant. “The only molecular system known to perform thermodynamically efficient water oxidation is PSII,” says Durrant. “We're a long way from having a molecular system that works as well,” he says.</p><p>Other chemists say that simply mimicking photosynthesis is too short sighted. “Photosynthesis is basically a failure for energy conversion,” says Tom Mallouk at Pennsylvania State University. To beat the world's energy problems, scientists have to be more ambitious than the 3% efficiency achieved by plants. “If that's all we want, the thing to do is grow corn,” says Mallouk. He thinks the goal should be at least 10%, preferably 20% power conversion efficiency, using materials that do not cost much more per unit area than house paint, he says.</p><p>The Powering the Planet team is optimistic it can beat photosynthesis, even if it doesn't beat Turner's efficiency record. And they are determined to beat Turner on cost. “In the end, we want to be at 5–10% solar-to-fuels efficiency,” says Lewis. “We know the materials that work. It's just a question of making it work faster, better, cheaper.”</p><p>Another materials approach to water splitting is being taken by Kazunari Domen at the University of Tokyo. In future, says Domen, if human beings use solar energy on a huge scale, we need to use technology that captures solar energy over much larger areas. To achieve this, he uses a photocatalyst particle that generates hydrogen and oxygen simultaneously on its own surface. The material is a solid solution, a mixture of metal oxides impregnated with nanoparticles of another mixed oxide. Domen is still developing these materials, which as yet don't work for all wavelengths of light.</p><p>25/53 0a3c5a2395ed61be11492a7dbf31b8fe.doc 2018/1/9</p><p>Domen admits there is still lots of basic research to be done. Indeed, all of the groups defend their work as basic chemistry first — and applied research second. “We're doing fundamental chemistry, unashamedly,” says Lewis. “There's lots of basic stuff to do to solve this.”</p><p>To get a fully practical system may take years, although Atwater thinks that the silicon-rod carpets are already ripe for commercialization as light-capturing solar panels. A few small companies are investigating water-splitting, including Nanoptek, a start-up firm in Maynard, Massachusetts, which is building on the original Kilby approach by developing photoelectrodes that will harvest photons over a wider energy range. </p><p>Fueling the future</p><p>G24 Innovations (G24i) in Cardiff, Wales, is developing small-scale electricity generating systems based on organic dyes — again mimicking nature's use of chlorophyll in photosynthesis — for personal electronics, mobile phones and laptops, with the goal of bringing these mass communication devices to remote parts of the world. The company's first products — solar-powered chargers — rolled off the production line in February.</p><p>The approach is based on technology pioneered by Michael Grätzel at the Swiss Federal Institute of Technology in Lausanne. In his photoelectrochemical cells, the amount of silicon needed is greatly reduced, because the expensive semiconductor is used purely to ferry electrons and holes around, and sunlight is captured by an organic dye. But G24i's products only generate electricity, rather than convert sunlight to fuel.</p><p>ADVERTISEMENT</p><p>There are different opinions in the field about when a commercial fuel-generating device will become reality. Where Atwater sees immediate commercial opportunities for his part of the project, Durrant can't see there being anything practical working for at least ten years. Gray thinks the problem requires at least another three or four years' work, and can see good motivation for pushing ahead. “This is a big deal in terms of competitiveness and innovation,” says Gray. “When solar energy comes in big time this is a trillion-dollar business.”</p><p>Turner wants to see a longer-term approach to pay for this kind of research. For the past 30 years, he says, funding has been “spotty and not focused”. This is a mistake, he thinks, if the future energy needs of the world are to be met. </p><p>26/53 0a3c5a2395ed61be11492a7dbf31b8fe.doc 2018/1/9</p><p>“Sunlight is truly our largest energy resource by far — it outshines everything else.” Published online 26 November 2008 | Nature 456, 436-440 (2008) | doi:10.1038/456436a </p><p>News Feature Car industry: Charging up the future</p><p>A new generation of lithium-ion batteries, coupled with rising oil prices and the need to address climate change, has sparked a global race to electrify transportation. Jeff Tollefson investigates.</p><p>Jeff Tollefson </p><p>Download a pdf of this story. "We have had a massive shift in one of the biggest industries in the world," says Stephan Dolezalek, who leads the CleanTech group at the venture-capital firm VantagePoint Venture Partners in San Bruno, California. Dolezalek has been watching the global automobile sector embrace the idea of plug-in electric cars: "In three years we've gone from thinking 'it can't be done' to not only 'it can be done' but 'we are all going to do it.'"</p><p>The shift is partly a story of technological innovation, which has produced rechargeable batteries that pack enough power to propel some of the basic passenger vehicles currently being designed further than 200 kilometres. Billions of dollars have poured into start-up companies that promise new batteries, and billions more have poured into fledgling electric-car manufacturers eager to take on the global automotive giants — every one of which is also developing electric vehicles.</p><p>The shift is also a story of oil supplies, national security and global warming. Record-high oil prices have pushed consumers towards fuel-efficient vehicles and prompted many governments to consider electric transport as a way to escape their dependence on imported petroleum and to address climate change. Money currently spent abroad could instead be spent on domestic power generation from wind, solar and other low-carbon energy sources. “Don't worry about charging electric cars from some perfect grid of the future – just get the cars out there.” Mark Duvall </p><p>27/53 0a3c5a2395ed61be11492a7dbf31b8fe.doc 2018/1/9</p><p>And the shift is a story of a shared vision: developing the technology that would entice all drivers to plug in rather than fill up. Millions of battery-powered cars plugged into an increasingly green electric grid would not only save drivers money and reduce greenhouse-gas emissions, it would also provide the grid with a distributed, high-capacity storage system for electricity. Such a system would help to accommodate the variable and unpredictable nature of renewable electricity sources. And further out, it could allow power companies to store energy generated during times of low demand, then draw it back again to meet peak demand. The end result could be more a stable and efficient grid that might even lower home electricity bills.</p><p>Getting there won't be easy. All these hopes hinge on battery technology that is only just emerging from the lab. A suite of technical challenges remains to be overcome, and it is not yet clear how much further the technology can be pushed. At the same time, the manufacturers who are arguably best able to bring about these changes — the global automotive giants — have been hammered by an energy crisis followed by an epic financial meltdown.</p><p>None of them has abandoned the effort yet, in large part because they all believe that, despite the current lull, oil prices have nowhere to go but up. Moreover, batteries have leapt ahead of expensive hydrogen fuel cells as the technology of choice for getting beyond oil, at least for now. But the field is wide open in terms of bringing them to market. Dolezalek believes that major car companies might well perish in the face of versatile young upstarts, and he isn't alone. The automobile industry secured a place in this autumn's first round of economic bailouts from the US government with US$25 billion in loan guarantees for retooling its plants, and it is already seeking more. That has people such as Andrew Grove, former chairman of Intel, who has become a leading proponent of electric transportation, talking about the 'valley of death' that often accompanies a massive technological transformation. Grove says that car manufacturers have already begun their march through the valley, knowing that many won't make it through to the other side.</p><p>"The only time people make these moves [through the valley] is when things are rough, but they can't afford to make them when times are rough," Grove says. And that means that governments might have to step in. "I just hope that it's going to be done in such a way that the government says, 'I'll give you some water and food to get through the valley of death, but don't turn back.'" </p><p>Building a better battery</p><p>Pioneers have turned back before, most notably General Motors. In 1996, the US company released the EV-1, the first all-electric car from a major manufacturer. The vehicle was expensive, rolled out in response to a California mandate, which was later rescinded, for 2% of all cars sold in the state to have zero-emissions by 1998. But its fate was ultimately sealed by one thing: its battery.</p><p>28/53 0a3c5a2395ed61be11492a7dbf31b8fe.doc 2018/1/9</p><p>Click for larger image. Building batteries has been an exercise in chemical compromise for more than two centuries. The idea is simple: chemical bonds can be used to trap ions in one electrode. When a battery is hooked up to a circuit, the ions flow through a separator to a second electrode; as the ions flow, they release electrons, generating an electric current. In rechargeable batteries, the chemical reaction can be reversed to store energy (see graphic, right). But the reality is complex: although scientists have produced numerous potential battery chemistries (see Nature 451, 652–657; 2008), none of them performs well on all the crucial factors of cost, safety, durability, power and sheer capacity.</p><p>The first-generation EV-1 deployed a lead-acid battery, still the technology of choice for conventional vehicles. Lead-acid batteries are safe, cheap, long-lived and reliable, but they are also big and heavy. They could push the car for about 150 kilometres per charge. A second-generation vehicle released in 1999 featured a nickel metal hydride battery, and travelled 50% farther on a charge, but General Motors cancelled them after the first year, saying that it could not sell enough to make them profitable.</p><p>The plug-in Prius uses electricity mostly to improve the efficiency of its petrol-powered motor.J. SULLIVAN/GETTY IMAGES</p><p>29/53 0a3c5a2395ed61be11492a7dbf31b8fe.doc 2018/1/9</p><p>It was a decision that General Motors would come to regret. As it turned back to large and profitable vehicles such as the Hummer, its up-and-coming Japanese rival Toyota was digging into the new technology, using the same battery that General Motors had abandoned to produce hybrid cars that combined a standard combustion engine with an electric motor. Toyota has gone on to set the standard for hybrids: its third-generation Prius has been immensely popular, proving that consumers will adopt advanced battery technology in automobiles if it is done well. The Prius fortified Toyota's reputation, and helped it to surpass General Motors last year to become the largest automobile manufacturer in the world.</p><p>But nickel metal hydride batteries can be developed only so far. These batteries pack more power than standard lead-acid ones but can be permanently damaged if allowed to discharge too far. To maintain an adequate safety margin, Toyota limited the Prius to using about 20% of its battery charge during normal operation. But although not using 80% of the capacity is acceptable if the battery is simply supplementing a petrol engine, it is a luxury that fully electric cars can't afford. Electric cars need all the charge they can get, and that means new chemistries.</p><p>Lithium-ion batteries, which are compact and have a high capacity, are a natural place to start. Sony paved the way with the lithium cobalt oxide battery, which made its mass-market debut in a 1991 version of the firm's HandyCam video camera, and is now widely used in consumer electronics. Lithium is a light metal, and the lithium cobalt oxide lattice structure allows plenty of space for the give and take of ions. But scaling this chemistry up for vehicles is problematic. Cobalt is expensive and toxic, and the batteries have been known to show 'thermal runaway', battery lingo for fires or explosions. "It has affected a tiny, tiny fraction of all of the batteries sold, but nonetheless, it's pretty freaky to think about a big fire in one of the vehicles," says Jeff Dahn, who works on advanced battery technology at Dalhousie University in Halifax, Canada. "Safety really needs to be the focus for the research community."</p><p>Many of the lithium batteries under development for vehicles replace cobalt oxides with manganese oxides and iron phosphates. Both are safer, but they do have their own problems, not least of which is a lower storage capacity for their size. Another challenge has been dealing with the physical expansion and contraction of the electrode material as the lithium ions flow back and forth during charge and discharge, which can lead to fractures. Researchers at multiple institutions have addressed the issue by adding carbon and other substances to the electrode material.</p><p>The Chevrolet Volt runs on electricity, with a petrol motor to help it go further.T. KIENZLE/AP They are also probing other chemistries — often at the nanoscale — based on silicon, fluorides and oxygen, which have a greater capacity. Others are looking at equipping the battery pack with capacitors, which can rapidly store and discharge electricity.</p><p>30/53 0a3c5a2395ed61be11492a7dbf31b8fe.doc 2018/1/9</p><p>Even in their current state, however, lithium-ion batteries are performing well enough to keep car manufacturers interested. Last year, General Motors inaugurated the race for mass-market electric vehicles when it announced plans to market its plug-in hybrid, the Chevrolet Volt. </p><p>A break with the past</p><p>The Volt, now scheduled for a 2010 roll-out, is a radical shift in design. Hybrids such as the Prius are powered by petrol, and use a battery simply to improve fuel efficiency. The Volt hybrid will be the reverse: an electric car that uses petrol to extend its range. Only when the charge dies will a small petrol motor kick in to charge the battery, which then continues to power the vehicle. The goal is for Volt owners to plug in at night and then drive more than 60 kilometres a day on a single charge — before burning a single drop of petrol. Given that as many as 80% of US drivers commute less than that on an average day, such vehicles could eliminate a sizeable chunk of the nation's oil consumption.</p><p>The Volt initiative could open the door to a new kind of transportation system — if the company can pull it off, both on time and at a cost that will tempt consumers. Many observers have their doubts. "They are fundamentally redefining what a car is, but can they do it? I don't know," says Don Hillebrand, who heads the Center for Transportation Research at Argonne National Laboratory in Illinois. "When the first generation of anything comes out, to a certain extent car manufacturers are rolling the dice, and this is the biggest roll of the dice anybody has ever made."</p><p>Some say it is a long shot. With sales plummeting in the midst of a deepening recession, the company is facing possible bankruptcy, and has joined with the other major US car manufacturers in seeking an additional bailout from the government. But through it all, General Motors has continued to sink everything it can spare into the Volt, viewing it as a key technology that would allow the company to leapfrog its competitors. “Safety really needs to be the focus for the research community.” Jeff Dahn </p><p>Toyota is taking a more measured approach with its plug-in hybrid, which is expected to roll out with a lithium-ion battery in 2009. John Hanson, a spokesman based at Toyota's US headquarters in Torrance, California, talks about managing customer expectations: the company is promising only that the vehicle will go "at least" 16 kilometres on an electric charge. After that, it will blend petrol and electric power in much the same way as the current Prius.</p><p>That would leave General Motors in pole position, at least in terms of the electric range it is promising. But will the Volt succeed? The answer to that question depends on consumers. What will they want several years from now? And how much will they be willing to pay? General Motors expects to lose money in the beginning and has not yet announced a price for the vehicle, but the continued viability of the firm could depend on how fast it can sell the new cars and at what price. The company is banking on tax credits, enacted this year by Congress, to encourage people to buy plug-in hybrids, and high petrol prices would help as well.</p><p>But the firm's chief economist Mustafa Mohatarem says that he can't help but wonder whether consumer demand for electric vehicles has been exaggerated. "It is critically dependent on the battery," he says. "Until you have a much better handle on the cost of this technology, to talk about demand is in a sense ridiculous." </p><p>31/53 0a3c5a2395ed61be11492a7dbf31b8fe.doc 2018/1/9</p><p>Have we forgotten something?</p><p>Others look at the market and see a different problem: a lack of batteries. Charles Gassenheimer, chief executive of Ener1 Group, a company in New York that produces lithium batteries, says that car manufacturers have collectively announced some 75 types of electric cars that are supposed to hit the road by 2013. But they have been slow to commit to orders, he says. And without orders, battery manufacturers can't invest the time and money necessary to ramp up production, a bottleneck that could delay the roll-out of new vehicles.</p><p>Governments seeking to spur the electric-car market must look at battery manufacturing in addition to consumers and car manufacturers, Gassenheimer says. "There needs to be some government intervention at this phase in the game. Otherwise it's going to be a chicken-and-egg problem that doesn't get solved."</p><p>Gassenheimer also raises concerns that countries such as the United States will simply trade their dependence on Middle Eastern oil for a reliance on Asian batteries. He has a sizeable stake in the outcome, of course, but the issue has political resonance as governments look to spur new green jobs. Experts say that Ener1 and other Western companies have the technology, but Asian companies have a leg-up on the manufacturing side simply because Asia has such a lead in producing lithium-ion batteries for electronics.</p><p>"The United States is certainly not being blindsided at this time, but whether or not we really have the resources and critical mass to compete in the long term in automotive batteries is still very much an open question," says Yet-Ming Chiang, a materials scientist at the Massachusetts Institute of Technology in Cambridge, and founder of lithium-battery manufacturer A123Systems in Watertown, Massachusetts. "The same thing goes for Europe." “We have some time to look at the next mega-application, and the next mega-application is the automotive industry.” Soonho Ahn </p><p>Others dismiss concerns about where the batteries are going to be made, citing a crucial difference between electronics and vehicles: electronics are by and large made in Asia, but cars are made in the West, too. Building batteries near automobile plants would not only save money, it would also get around complex international shipping regulations that put lithium-ion batteries in the 'dangerous goods' category. The market "is driven by where the end product is", says Khalil Amine, a battery researcher and one of Hillebrand's colleagues at Argonne. "For electronics, we buy everything from Asia. For transportation, there is plenty of production here."</p><p>General Motors tested lithium batteries from every manufacturer it could find and narrowed the decision down to two companies: A123Systems and LG Chem, a Korean giant that made its name in electronics. Only in late October did the contract reportedly go to LG Chem, which has a stronger base and a longer history on the manufacturing side. LG Chem has already partnered with the Korean car manufacturer Hyundai to supply 7,000–10,000 lithium-battery packs for a pair of hybrid vehicles that will begin rolling off the line in 2009.</p><p>Soonho Ahn, LG Chem's vice-president for battery research and development, says that his company isn't expecting to make money on its automotive batteries for some time but wants to be ready when the market takes off. He notes that the battery market in Asia is in "equilibrium" after several years of stiff competition in the electronics sector. "We have some time to look at the next </p><p>32/53 0a3c5a2395ed61be11492a7dbf31b8fe.doc 2018/1/9 mega-application, and the next mega-application is the automotive industry," he says. "We're pretty sure that the market is coming." </p><p>Making connections</p><p>So what will the market be like when it does come? Plug-in hybrids such as the Volt represent a leap beyond battery-augmented cars that merely make better use of petrol. They also give drivers the freedom to run on electricity for short trips while still making long trips, albeit guzzling gas on the way. But some car manufacturers say that the best path forward would be an all-electric vehicle, which could one day all but eliminate oil consumption in the transportation sector.</p><p>Getting rid of the petrol motor greatly lessens costs and complexity and opens up space for more battery power. "In terms of a solution, both from a carbon dioxide point of view and from a technical point of view, the hybrid and the plug-in hybrid do not provide the technical breakthrough that the electric vehicle could provide," says Serge Yoccoz, who is in charge of electric vehicles at Renault. "And from what we've seen, the plug-in hybrid is definitely more expensive [than an electric car would be], even if you take into account the need to develop a charging infrastructure."</p><p>So while researchers search for the technical breakthrough, entrepreneurs are trying to get around the high costs by rethinking the way we market cars, batteries and ultimately energy.</p><p>Click for larger image. One such innovator, Better Place of Palo Alto, California, is aiming for nothing short of a wholesale conversion of the transportation sector. The company likens itself to a cell-phone network for all-electric cars: you buy the car from a Better Place partner and then sign up for one of its various user plans. Better Place then provides a network of charging spots — at home, work and retail outlets — as well as stations at which used battery packs could be swapped for recharged ones by a robotic arm in a matter of minutes (see graphic, right).</p><p>But to accomplish all this, Better Place needs a computer system that can track electricity charges wherever they are incurred. It also needs to partner with governments and industry, including the automotive, battery and utility sectors. So far, Better Place has lined up partnerships with an alliance between Nissan and Renault to pursue electric cars, and the company plans to roll out its system in </p><p>33/53 0a3c5a2395ed61be11492a7dbf31b8fe.doc 2018/1/9</p><p>Israel, Denmark, Australia and California, with the first deployments scheduled for 2010.</p><p>The scheme is ambitious, but Sidney Goodman, head of automotive alliances at Better Place, says that's the only way to do it. "We don't believe we can do this on a small scale. It's one of these projects where either you do it big or you don't do it".</p><p>Better Place is aiming to provide family sedans that have a 160-kilometre range in an effort to attract all drivers, not just city commuters with an environmental bent. Goodman runs through some rough numbers — assuming that a battery costs US$15,000 (which is likely to be on the high end of the scale, he stresses), an electric vehicle would cost about 6 cents per kilometre to power. That compares with just under 12 cents per kilometre for conventional cars in the United States, and twice that in Europe.</p><p>A Norwegian company called Th!nk is taking a similar route with its all-electric commuter car, which is due to hit roads in Norway, Denmark and Sweden in coming months. With an initial price tag of about 200,000 Norwegian krone ($30,000), the car will cost about 20% more than the same-sized petrol-powered car and will drive some 180 kilometres on a charge. Customers then pay a monthly lease to cover the cost of electricity and the battery. "We'll get the costs of our car down to somewhat similar to the cost of a petrol-powered car, and then we'll have a very strong proposition going forwards," says Richard Canny, Th!nk's chief executive. </p><p>Tapping the matrix</p><p>Utility firms are eager to cooperate. Although making electric vehicles a reality will require unprecedented cooperation between two industries that have until now had little in common, utilities actually see many more benefits than headaches. The fundamental fact is that most of the charging would take place at night, which creates a new source of revenue at a time when utilities typically have excess capacity.</p><p>In the end, this should translate into substantial reductions in greenhouse-gas emissions, even in countries such as the United States that get much of their electricity from coal. A plug-in hybrid running on electricity generated entirely from coal is roughly equivalent to a conventional hybrid in terms of emissions, but utilities say that in the early years, electric vehicles will frequently draw power from spare generating capacity that uses cleaner-burning natural gas. Scaled up, millions of batteries — either in cars or in a future after-market for used batteries — could provide utilities with a flexible storage system that could soak up renewable power, particularly from wind turbines at night.</p><p>Assuming that plug-in hybrids will make up 60% of the US automobile market in 2050, electric transport would consume as little as 8% of the nation's electricity, according to a joint modelling study conducted in 2007 by the Electric Power Research Institute — a non-profit research organization in Palo Alto — and the Natural Resources Defense Council, an environmental advocacy group based in </p><p>New York. The resulting report, The Power to Reduce CO2 Emissions, predicts that the nation would use 15–20% less oil and reduce its greenhouse-gas emissions by 450 million tonnes, which is akin to pulling 82.5 million internal-combustion vehicles off the road.</p><p>"Our fundamental conclusion from this study is that the number one driver of benefits is really the number of vehicles," says Mark Duvall, programme manager</p><p>34/53 0a3c5a2395ed61be11492a7dbf31b8fe.doc 2018/1/9 of electric transportation at the Electric Power Research Institute. "Don't worry about charging them from some perfect grid of the future – just get the cars out there. They don't have to be perfect."</p><p>Utilities such as Southern California Edison in Rosemead are already thinking about how to integrate cars into the electricity system, allowing them to charge up at work or park in 'smart garages' that coordinate activities between the car and the grid. In the early days, advanced charging equipment would communicate with the utility to time the charging so that everybody's vehicle is fully juiced when it needs to be — but not necessarily before. That would help ensure that millions of vehicles don't create a sudden surge on the electricity system when people return from work, when they also tend to turn on lights and crank up their appliances. Further out, this process could be reversed, allowing batteries to provide power to the grid when it is needed most — so long as they are fully charged when it comes to time to drive.</p><p>ADVERTISEMENT</p><p>Levelling out the daily demand cycles would allow utilities to manage the grid more efficiently, potentially lowering costs to consumers. "The more cars that come onto the energy system, the better off it is for the energy system," says Ed Kjaer, director of electric transportation for Southern California Edison. And Kjaer says that the vehicles will become cleaner over time as utilities expand their renewable electricity offerings.</p><p>That kind of logic has convinced many researchers that electric cars are a must if the planet is to deal with global warming, even if they ultimately raise the stakes on efforts to produce carbon-free electricity. "We've got to electrify the transportation system and then clean up the grid," says Timothy Lipman, research director at the University of California's Transportation Sustainability Research Center in Berkeley. "It's the easiest path."</p><p>See Editorial, page 421 See also Correspondence: Choosing between batteries or biomass to stay on the road</p><p>Comments</p><p>Reader comments are usually moderated after posting. If you find something offensive or inappropriate, you can speed this process by clicking 'Report this comment' (or, if that </p><p>35/53 0a3c5a2395ed61be11492a7dbf31b8fe.doc 2018/1/9</p><p> doesn't work for you, email [email protected]). For more controversial topics, we reserve the right to moderate before comments are published.</p><p> Very interesting article on electric vehicles. Too bad that the clunky looking Chevy Volt was the head-liner. It still has an internal combustion engine! Not one mention of an all-electric, 200 mile range vehicle that is already on the road - the Tesla Roadster, soon to be followed by the White Star Sedan - from the same company in San Carlos California! Imagine if that company got some additional financing from the government to move more rapidly into the general market, and out of the expensive boutique market. Their slick looking electrics would be common place on the daily commuter trip! And this would be going on without all of the dripping oil, fluids, and crap into the air. It is easier to control pollution at the stationary source of electric generation than on a billion mobile polluting units called cars. One of many obstacles to getting that block of oil burning metal out of American cars - the UAW! Just think for a moment: Making a vehicle with 5 moving parts that runs on electricity as opposed to making internal combustion engines and drive-line with hundreds of parts would eliminate a lot of union jobs! What about the after market racket on spare parts? I believe that much innovation in our auto industry has been stifled by a union strangle-hold, and that there has been little incentive to change the status quo. Electrics won't replace the heavy lifting to be done by trucks, but as an alternative to the millions of folks sitting in their 6,000 lb. 20 ft. long SUVs, the daily commute in an electric makes some sense for me. Soarhead o Report this comment o 01 Dec, 2008 o Posted by: Gary Filice Published online 13 August 2008 | Nature 454, 816-823 (2008) | doi:10.1038/454816a </p><p>News Feature Energy alternatives: Electricity without carbon</p><p>Quirin Schiermeier , Jeff Tollefson , Tony Scully , Alexandra Witze & Oliver Morton </p><p>This article is best viewed as a PDF.</p><p>Electricity generation provides 18,000 terawatt-hours of energy a year, around 40% of humanity's total energy use. In doing so it produces more than 10 gigatonnes of carbon dioxide every year, the largest sectoral contribution of humanity's fossil-fuel derived emissions. Yet there is a wide range of technologies — from solar and wind to nuclear and geothermal — that can generate electricity without net carbon emissions from fuel. </p><p>The easiest way to cut the carbon released by electricity generation is to increase efficiency. But there are limits to such gains, and there is the familiar paradox that greater efficiency can lead to greater consumption. So a global response to climate change must involve a move to carbon- free sources of electricity. This requires fresh thinking about the price of carbon, and in some cases new technologies; it also means new transmission systems and smarter grids. But above all, the various sources of carbon-free generation need to be scaled up to power an increasingly demanding world. In this special feature, Nature's News team looks at how much carbon-free energy might ultimately be available — and which sources make most sense. </p><p>36/53 0a3c5a2395ed61be11492a7dbf31b8fe.doc 2018/1/9</p><p>Hydropower</p><p>J. TAYLOR The world has a lot of dams — 45,000 large ones, according to the World Energy Council, and many more at small scales. Its hydroelectric power plants have a generating capacity of 800 gigawatts (for a guide to power, see ‘By the numbers’), and they currently supply almost one-fifth of the electricity consumed worldwide. As a source of electricity, dams are second only to fossil fuels, and generate 10 times more power than geothermal, solar and wind power combined. With a claimed full capacity of 18 gigawatts, the Three Gorges dam in China can generate more or less twice as much power as all the world's solar cells. An additional 120 gigawatts of capacity is under development.</p><p>One reason for hydropower's success is that it is a widespread resource — 160 countries use hydropower to some extent. In several countries hydropower is the largest contributor to grid electricity — it is not uncommon in developing countries for a large dam to be the main generating source. Nevertheless, it is in large industrialized nations that have big rivers that hydroelectricity is shown in its most dramatic aspect. Brazil, Canada, China, Russia and the United States currently produce more than half of the world's hydropower.</p><p>Cost: According to the International Hydropower Association (IHA), installation costs are usually in the range of US$1 million to more than $5 million per megawatt of capacity, depending on the site and size of the plant. Dams in lowlands and those with only a short drop between the water level and the turbine tend to be more expensive; large dams are cheaper per watt of capacity than small dams in similar settings. Annual operating costs are low — 0.8–2% of capital costs; electricity costs $0.03–0.10 per kilowatt-hour, which makes dams competitive with coal and gas.</p><p>Capacity: The absolute limit on hydropower is the rate at which water flows downhill through the world's rivers, turning potential energy into kinetic energy </p><p>37/53 0a3c5a2395ed61be11492a7dbf31b8fe.doc 2018/1/9 as it goes. The amount of power that could theoretically be generated if all the world's run-off were 'turbined' down to sea level is more than 10 terawatts. However, it is rare for 50% of a river's power to be exploitable, and in many cases the figure is below 30%.</p><p>Those figures still offer considerable opportunity for new capacity, according to the IHA. Europe currently sets a benchmark for hydropower use, with 75% of what is deemed feasible already exploited. For Africa to reach the same level, it would need to increase its hydropower capacity by a factor of 10 to more than 100 gigawatts. Asia, which already has the greatest installed capacity, also has the greatest growth potential. If it were to triple its generating capacity, thus harnessing a near-European fraction of its potential, it would double the world's overall hydroelectric capacity. The IHA says that capacity could triple worldwide with enough investment.</p><p>Advantages: The fact that hydroelectric systems require no fuel means that they also require no fuel-extracting infrastructure and no fuel transport. This means that a gigawatt of hydropower saves the world not just a gigawatt's worth of coal burned at a fossil-fuel plant, but also the carbon costs of mining and transporting that coal. As turning on a tap is easy, dams can respond almost instantaneously to changing electricity demand independent of the time of day or the weather. This ease of turn-on makes them a useful back-up to less reliable renewable sources. That said, variations in use according to need and season mean that dams produce about half of their rated power capacity.</p><p>Hydroelectric systems are unique among generating systems in that they can, if correctly engineered, store the energy generated elsewhere, pumping water uphill when energy is abundant. The reservoirs they create can also provide water for irrigation, a way to control floods and create amenities for recreational use.</p><p>Disadvantages: Not all regions have large hydropower resources — the Middle East, for example, is relatively deficient. And reservoirs take up a lot of space; today the area under man-made lakes is as large as two Italys. The large dams and reservoirs that account for most of that area and for more than 90% of hydro-generated electricity worldwide require lengthy and costly planning and construction, as well as the relocation of people from the reservoir area. In the past few decades, millions of people have been relocated in India and China. Dams have ecological effects on the ecosystems upstream and downstream, and present a barrier to migrating fish. Sediment build-up can shorten their operating life, and sediment trapped by the dam is denied to those downstream. Biomass that decomposes in reservoirs releases methane and carbon dioxide, and in some cases these emissions can be of a similar order of magnitude to those avoided by not burning fossil fuels. Climate change could itself limit the capacity of dams in some areas by altering the amount and pattern of annual run-off from sources such as the glaciers of Tibet.</p><p>Because hydro is a mature technology, there is little room for improvement in the efficiency of generation. Also, the more obvious and easy locations have been used, and so the remaining potential can be expected to be harder to exploit. Small (less than 10 megawatts) 'run-of-river' schemes that produce power from the natural flow of water — as millers have been doing for four millennia — are appealing, as they have naturally lower impacts. However, they are about five times more expensive and harder to scale than larger schemes.</p><p>Verdict: A cheap and mature technology, but with substantial environmental costs; roughly a terawatt of capacity could be added. </p><p>38/53 0a3c5a2395ed61be11492a7dbf31b8fe.doc 2018/1/9</p><p>Nuclear fission</p><p>J. TAYLOR When reactor 4 at the Chernobyl nuclear power plant in Ukraine melted down on 26 April 1986, the fallout contaminated large parts of Europe. That disaster, and the earlier incident at Three Mile Island in Pennsylvania, blighted the nuclear industry in the West for a generation. Worldwide, though, the picture did not change quite as dramatically.</p><p>In 2007, 35 nuclear plants were under construction, almost all in Asia. The 439 reactors already in operation had an overall capacity of 370 gigawatts, and contributed around 15% of the electricity generated worldwide, according to the most recent figures from the International Atomic Energy Agency (IAEA), which serves as the world's nuclear inspectorate.</p><p>Costs: Depending on the design of the reactor, the site requirements and the rate of capital depreciation, the light-water reactors that make up most of the world's nuclear capacity produce electricity at costs of between US$0.025 and $0.07 per kilowatt-hour. The technology that makes this possible has benefited from decades of expensive research, development and purchases subsidized by governments; without that boost it is hard to imagine that nuclear power would currently be in use.</p><p>Capacity: Because nuclear power requires fuel, it is constrained by fuel stocks. There are some 5.5 million tonnes of uranium in known reserves that could profitably be extracted at a cost of US$130 per kilogram or less, according to the latest edition of the 'Red Book', in which the IAEA and the Organisation for Economic Co-operation and Development (OECD) assess uranium resources. At the current use of 66,500 tonnes per year, that is about 80 years' worth of fuel. The current price of uranium is over that $130 threshold.</p><p>Geologically similar ore deposits that are as yet unproven — 'undiscovered reserves' — are thought to amount to roughly double the proven reserves, and lower-grade ores offer considerably more. Uranium is not a particularly rare element — it is about as common a constituent of Earth's crust as zinc. Estimates of the ultimate recoverable resource vary greatly, but 35 million tonnes might be considered available. Nor is uranium the only naturally occurring element that can be made into nuclear fuel. Although they have not yet been developed, thorium- fuelled reactors are a possibility; bringing thorium into play would double the available fuel reserves.</p><p>39/53 0a3c5a2395ed61be11492a7dbf31b8fe.doc 2018/1/9</p><p>Furthermore, although current reactor designs use their fuel only once, this could be changed. Breeder reactors, which make plutonium from uranium isotopes that are not themselves useful for power production, can effectively create more fuel than they use. A system built on such reactors might get 60 times more energy out for every kilogram of natural uranium put in, although lower multiples might be more realistic.</p><p>With breeder reactors, which have yet to be proven on a commercial basis, the world could in principle go 100% nuclear. Without them, it is still plausible for the amount of nuclear capacity to grow by a factor of two or three, and to operate at that level for a century or more.</p><p>Advantages: Nuclear power has relatively low fuel costs and can run at full blast almost constantly — US plants deliver 90% of their rated capacity. This makes them well suited to providing always-on 'baseload' power to national grids. Uranium is sufficiently widespread that the world's nuclear-fuel supply is unlikely to be threatened by political factors.</p><p>Disadvantages: There is no agreed solution to the problem of how to deal with the nuclear waste that has been generated in nuclear plants over the past 50 years. Without long-term solutions, which are more demanding politically than technically, growth in nuclear power is an understandably hard sell. A further problem is that the spread of nuclear power is difficult to disentangle from the proliferation of nuclear weapons capabilities. Fuel cycles that involve recycling, and which thus necessarily produce plutonium, are particularly worrying. Even without proliferation worries, nuclear power stations may make tempting targets for terrorists or enemy forces (although in the latter case the same is true of hydroelectric plants).</p><p>A long-term commitment to greatly increased use of nuclear power would require public acceptance not just of existing technologies but of new ones, too — thorium and breeder reactors, for instance. These technologies would also have to win over investors and regulators (for nuclear fusion, see ‘Farther out’).</p><p>Nuclear power is also extremely capital intensive; power costs over the life of the plant are comparatively low only because the plants are long lived. Nuclear power is thus an expensive option in the short term. Another constraint may be a lack of skilled workers. Building and operating nuclear plants requires a great many highly trained professionals, and enlarging this pool of talent enough to double the rate at which new plants are brought online might prove very challenging. The engineering capacity for making key components would also need enlarging.</p><p>In light of these obstacles, predictions of the future role of nuclear power vary considerably. The European Commission's World Energy Technology Outlook — 2050 contains a bullish scenario that assumes that, with public acceptance and the development of new reactor technologies, nuclear power could provide about 1.7 terawatts by 2050. The IAEA's analysts are more cautious. Hans-Holger Rogner, head of the agency's planning and economic study section, sees capacity rising to not more than 1,200 gigawatts by 2050. An interdisciplinary study carried out in 2003 by the Massachusetts Institute of Technology described a concrete scenario for tripling capacity to 1,000 gigawatts by 2050, a scenario predicated on US leadership, continued commitment by Japan and renewed activity by Europe. This scenario relied only on improved versions of today's reactors rather than on any radically different or improved design.</p><p>Verdict: Reaching a capacity in the terawatt range is technically possible over the next few decades, but it may be difficult politically. A climate of opinion that </p><p>40/53 0a3c5a2395ed61be11492a7dbf31b8fe.doc 2018/1/9 came to accept nuclear power might well be highly vulnerable to adverse events such as another Chernobyl-scale accident or a terrorist attack. </p><p>Biomass</p><p>J. TAYLOR Biomass was humanity's first source of energy, and until the twentieth century it remained the largest; even today it comes second only to fossil fuels. Wood, crop residues and other biological sources are an important energy source for more than two billion people. Mostly, this fuel is burned in fires and cooking stoves, but over recent years biomass has become a source of fossil-fuel-free electricity. As of 2005, the World Energy Council estimates biomass generating capacity to be at least 40 gigawatts, larger than any renewable resource other than wind and hydropower. Biomass can supplement coal or in some cases gas in conventional power plants. Biomass is also used in many co-generation plants that can capture 85–90% of the available energy by making use of waste heat as well as electric power.</p><p>Costs: The price of biomass electricity varies widely depending on the availability and type of the fuel and the cost of transporting it. Capital costs are similar to those for fossil-fuel plants. Power costs can be as little as $0.02 per kilowatt-hour when biomass is burned with coal in a conventional power plant, but increase to $0.03–0.05 per kilowatt-hour from a dedicated biomass power plant. Costs increase to $0.04–0.09 per kilowatt-hour for a co-generation plant, but recovery and use of the waste heat makes the process much more efficient. The biggest problem for new biomass power plants is finding a reliable and concentrated feedstock that is available locally; keeping down transportation costs means keeping biomass power plants tied to locally available fuel and quite small, which increases the capital cost per megawatt.</p><p>Capacity: Biomass is limited by the available land surface, the efficiency of photosynthesis, and the supply of water. An OECD round table in 2007 estimated that there is perhaps half a billion hectares of land not in agricultural use that would be suitable for rain-fed biomass production, and suggested that by 2050 this land, plus crop residues, forest residues and organic waste might provide enough burnable material each year to provide 68,000 terawatt-hours. Converted to electricity at an efficiency of 40%, that could provide a maximum of 3 </p><p>41/53 0a3c5a2395ed61be11492a7dbf31b8fe.doc 2018/1/9 terawatts. The Intergovernmental Panel on Climate Change pegs the potential at roughly 120,000 terawatt-hours in 2050, which equates to slightly more than 5 terawatts on the basis of a larger estimate of available land.</p><p>These projections involve some fairly extreme assumptions about converting land to the production of energy crops. And even to the extent that these assumptions prove viable, electricity is not the only potential use for such plantations. By storing solar energy in the form of chemical bonds, biomass lends itself better than other renewable energy resources to the production of fuel for transportation (see page 841). Although turning biomass to biofuel is not as efficient as just burning the stuff, it can produce a higher-value product. Biofuels might easily beat electricity generation as a use for biomass in most settings.</p><p>Advantages: Plants are by nature carbon-neutral and renewable, although agriculture does use up resources, especially if it requires large amounts of fertilizer. The technologies needed to burn biomass are mature and efficient, especially in the case of co-generation. Small systems using crop residues can minimize transportation costs.</p><p>If burned in power plants fitted with carbon-capture-and-storage hardware, biomass goes from being carbon neutral to carbon negative, effectively sucking carbon dioxide out of the atmosphere and storing it in the ground (see 'Carbon capture and storage'). This makes it the only energy technology that can actually reduce carbon dioxide levels in the atmosphere. As with coal, however, there are costs involved in carbon capture, both in terms of capital set-up and in terms of efficiency.</p><p>Disadvantages: There is only so much land in the world, and much of it will be needed to provide food for the growing global population. It is not clear whether letting market mechanisms drive the allocation of land between fuel and food is desirable or politically feasible. Changing climate could itself alter the availability of suitable land. There is likely to be opposition to increased and increasingly intense cultivation of energy crops. Use of waste and residues may remove carbon from the land that would otherwise have enriched the soil; long-term sustainability may not be achievable.</p><p>Bioenergy dependence could also open the doors to energy crises caused by drought or pestilence, and land-use changes can have climate effects of their own: clearing land for energy crops may produce emissions at a rate the crops themselves are hard put to offset.</p><p>Verdict: If a large increase in energy crops proves acceptable and sustainable, much of it may be used up in the fuel sector. However, small-scale systems may be desirable in an increasing number of settings, and the possibility of carbon- negative systems — which are plausible for electricity generation but not for biofuels — is a unique and attractive capability. </p><p>Wind</p><p>42/53 0a3c5a2395ed61be11492a7dbf31b8fe.doc 2018/1/9</p><p>J. TAYLOR Wind power is expanding faster than even its fiercest advocates could have wished a few years ago. The United States added 5.3 gigawatts of wind capacity in 2007 — 35% of the country's new generating capacity — and has another 225 gigawatts in the planning stages. There is more wind-generating capacity being planned in the United States than for coal and gas plants combined. Globally, capacity has risen by nearly 25% in each of the past five years, according to the Global Wind Energy Council.</p><p>Wind Power Monthly estimates that the world's installed capacity for wind as of January 2008 was 94 gigawatts. If growth continued at 21%, that figure would triple over six years.</p><p>Despite this, the numbers remain small on a global scale, especially given that wind farms have historically generated just 20% of their capacity.</p><p>Costs: Installation costs for wind power are around US$1.8 million per megawatt for onshore developments and between $2.4 million and $3 million for offshore projects. That translates to $0.05–0.09 per kilowatt-hour, making wind competitive with coal at the lower end of the range. With subsidies, as enjoyed in many countries, the costs come in well below those for coal — hence the boom. The main limit on wind-power installation at the moment is how fast manufacturers can make turbines.</p><p>These costs represent significant improvements in the technology. In 1981, a wind farm might have consisted of an array of 50-kilowatt turbines that produced power for roughly $0.40 per kilowatt-hour. Today's turbines can produce 30 times as much power at one-fifth the price with much less down time.</p><p>Capacity: The amount of energy generated by the movement of Earth's atmosphere is vast — hundreds of terawatts. In a 2005 paper, a pair of researchers from Stanford University calculated that at least 72 terawatts could be effectively generated using 2.5 million of today's larger turbines placed at the 13% of locations around the world that have wind speeds of at least 6.9 metres per second and are thus practical sites ( C. L. Archer and M. Z. Jacobson J. Geophys. Res. 110, D12110; 2005).</p><p>Advantages: The main advantage of wind is that, like hydropower, it doesn't need fuel. The only costs therefore come from building and maintaining the turbines and power lines. Turbines are getting bigger and more reliable. The development of technologies for capturing wind at high altitudes could provide sources with small footprints capable of generating power in a much more sustained way.</p><p>43/53 0a3c5a2395ed61be11492a7dbf31b8fe.doc 2018/1/9</p><p>Disadvantages: Wind's ultimate limitation might be its intermittency. Providing up to 20% of a grid's capacity from wind is not too difficult. Beyond that, utilities and grid operators need to take extra steps to deal with the variability. Another grid issue, and one that is definitely limiting in the near term, is that the windiest places are seldom the most populous, and so electricity from the wind needs infrastructure development — especially for offshore settings.</p><p>Average power of the world's winds during the boreal winter (top) and summer. The recoupable energy is some two orders of magnitude lower because of turbine spacing and engineering constraints. Courtesy: W. T. Liu et al. Geophys. Res. Lett. 35, L13808 (2008). As well as being intermittent, wind power is, like other renewable energy sources, inherently quite low density. A large wind farm typically generates a few watts per square metre — 10 is very high. Wind power thus depends on cheap land, or on land being used for other things at the same time, or both. It is also hard to deploy in an area where the population sets great store by the value of a turbine- free landscape.</p><p>Wind power is also unequally distributed: it favours nations with access to windy seas and their onshore breezes or great empty plains. Germany has covered much of its windiest land with turbines, but despite these pioneering efforts, its combined capacity of 22 GW supplies less than 7% of the country's electricity needs. Britain, which has been much slower to adopt wind power, has by far the largest offshore potential in Europe — enough to meet its electricity needs three times over, according to the British Wind Energy Association. Industry estimates suggest that the European Union could meet 25% of its current electricity needs by developing less than 5% of the North Sea.</p><p>Such truly large-scale deployment of wind-power schemes could affect local, and potentially global, climate by altering wind patterns, according to research by David Keith, head of the Energy and Environmental Systems Group at the University of Calgary in Canada. Wind tends to cool things down, so temperatures around a very large wind farm could rise as turbines slow the wind to extract its energy. Keith and his team suggest that 2 TW of wind capacity could affect temperatures by about 0.5 °C, with warming at mid-latitudes and cooling at the poles — perhaps in that respect offsetting the effect of global warming (D. W. Keith et al . Proc. Natl Acad. Sci. USA 101, 16115–16120; 2004).</p><p>Verdict: With large deployments on the plains of the United States and China, and cheaper access to offshore, a wind-power capacity of a terawatt or more is plausible. </p><p>44/53 0a3c5a2395ed61be11492a7dbf31b8fe.doc 2018/1/9</p><p>Geothermal</p><p>J. TAYLOR Earth's interior contains vast amounts of heat, some of it left over from the planet's original coalescence, some of it generated by the decay of radioactive elements. Because rock conducts heat poorly, the rate at which this heat flows to the surface is very slow; if it were quicker, Earth's core would have frozen and its continents ceased to drift long ago.</p><p>The slow flow of Earth's heat makes it a hard resource to use for electricity generation except in a few specific places, such as those with abundant hot springs. Only a couple of dozen countries produce geothermal electricity, and only five of those — Costa Rica, El Salvador, Iceland, Kenya and the Philippines — generate more than 15% of their electricity this way. The world's installed geothermal electricity capacity is about 10 gigawatts, and is growing only slowly — about 3% per year in the first half of this decade. A decade ago, geothermal capacity was greater than wind capacity; now it is almost a factor of ten less.</p><p>Earth's heat can also be used directly. Indeed, small geothermal heat pumps that warm houses and businesses directly may represent the greatest contribution that Earth's warmth can make to the world's energy budget.</p><p>Costs: The cost of a geothermal system depends on the geological setting. Jefferson Tester, a chemical engineer who was part of a team that produced an influential Massachusetts Institute of Technology (MIT) report on geothermal technology in 2006, explains the situation as being “similar to mineral resources. There is a continuum of resource grades — from shallow, high-temperature regions of high-porosity rock, to deeper low-porosity regions that are more challenging to exploit”. That report put the cost of exploiting the best sites — those with a lot of hot water circulating close to the surface — at about US$0.05 per kilowatt-hour. Much more abundant low-grade resources are exploitable with current technology only at much higher prices.</p><p>45/53 0a3c5a2395ed61be11492a7dbf31b8fe.doc 2018/1/9</p><p>Absolute capacity: Earth loses heat at between 40 TW and 50 TW a year, which works out at an average of a bit less than a tenth of a watt per square metre. For comparison, sunlight comes in at an average of 200 watts per square metre. With today's technology, 70 GW of the global heat flux is seen as exploitable. With more advanced technologies, at least twice that could be used. The MIT study suggested that using enhanced systems that inject water at depth using sophisticated drilling systems, it would be possible to set up 100 GW of geothermal electricity in the United States alone. With similar assumptions a global figure of a terawatt or so can be reached, suggesting that geothermal could, with a great deal of investment, provide as much electricity as dams do today.</p><p>Advantages: Geothermal resources require no fuel. They are ideally suited to supplying base-load electricity, because they are driven by a very regular energy supply. At 75%, geothermal sources boast a higher capacity factor than any other renewable. Low-grade heat left over after generation can be used for domestic heating or for industrial processes.</p><p>Surveying and drilling previously unexploited geothermal resources has become much easier thanks to mapping technology and drilling equipment designed by the oil industry. A significant technology development programme — Tester suggests $1 billion over 10 years — could greatly expand the achievable capacity as lower-grade resources are opened up.</p><p>Disadvantages: High-grade resources are quite rare, and even low-grade resources are not evenly distributed. Carbon dioxide can leak out of some geothermal fields, and there can be contamination issues; the water that brings the heat to the surface can carry compounds that shouldn't be released into aquifers. In dry regions, water availability can be a constraint. Large-scale exploitation requires technologies that, although plausible, have not been demonstrated in the form of robust, working systems.</p><p>Verdict: Capacity might be increased by more than an order of magnitude. Without spectacular improvements, it is unlikely to outstrip hydro and wind and reach a terawatt. </p><p>Solar</p><p>J. TAYLOR Not to take anything away from the miracle of photosynthesis, but even under the best conditions plants can only turn about 1% of the solar radiation that hits their surfaces into energy that anyone else can use. For comparison, a standard commercial solar photovoltaic panel can convert 12–18% of the energy of sunlight into useable electricity; high-end models come in above 20% efficiency. Increasing manufacturing capacity and decreasing costs have led to remarkable </p><p>46/53 0a3c5a2395ed61be11492a7dbf31b8fe.doc 2018/1/9 growth in the industry over the past five years: in 2002, 550 MW of cells were shipped worldwide; in 2007 the figure was six times that. Total installed solar-cell capacity is estimated at 9 GW or so. The actual amount of electricity generated, though, is considerably less, as night and clouds decrease the power available. Of all renewables, solar currently has the lowest capacity factor, at about 14%.</p><p>Solar cells are not the only technology by which sunlight can be turned into electricity. Concentrated solar thermal systems use mirrors to focus the Sun's heat, typically heating up a working fluid that in turn drives a turbine. The mirrors can be set in troughs, in parabolas that track the Sun, or in arrays that focus the heat on a central tower. As yet, the installed capacity is quite small, and the technology will always remain limited to places where there are a lot of cloud-free days — it needs direct sun, whereas photovoltaics can make do with more diffuse light.</p><p>Costs: The manufacturing cost of solar cells is currently US$1.50–2.50 for a watt's worth of generating capacity, and prices are in the $2.50–3.50 per watt range. Installation costs are extra; the price of a full system is normally about twice the price of the cells. What this means in terms of cost per kilowatt-hour over the life of an installation varies according to the location, but it comes out at around $0.25–0.40. Manufacturing costs are dropping, and installation costs will also fall as photovoltaic cells integrated into building materials replace free- standing panels for domestic applications. Current technologies should be manufacturing at less than $1 per watt within a few years (see Nature 454, 558– 559; 2008).</p><p>The cost per kilowatt-hour of concentrated solar thermal power is estimated by the US National Renewable Energy Laboratory (NREL) in Golden, Colorado, at about $0.17.</p><p>Capacity: Earth receives about 100,000 TW of solar power at its surface — enough energy every hour to supply humanity's energy needs for a year. There are parts of the Sahara Desert, the Gobi Desert in central Asia, the Atacama in Peru or the Great Basin in the United States where a gigawatt of electricity could be generated using today's photovoltaic cells in an array 7 or 8 kilometres across. Theoretically, the world's entire primary energy needs could be served by less than a tenth of the area of the Sahara.</p><p>Advocates of solar cells point to a calculation by the NREL claiming that solar panels on all usable residential and commercial roof surfaces could provide the United States with as much electricity per annum as the country used in 2004. In more temperate climes things are not so promising: in Britain one might expect an annual insolation of about 1,000 kilowatt-hours per metre on a south-facing panel tilted to take account of latitude: at 10% efficiency, that means more than 60 square metres per person would be needed to meet current UK electricity consumption.</p><p>Advantages: The Sun represents an effectively unlimited supply of fuel at no cost, which is widely distributed and leaves no residue. The public accepts solar technology and in most places approves of it — it is subject to less geopolitical, environmental and aesthetic concern than nuclear, wind or hydro, although extremely large desert installations might elicit protests.</p><p>Photovoltaics can often be installed piecemeal — house by house and business by business. In these settings, the cost of generation has to compete with the retail price of electricity, rather than the cost of generating it by other means, which gives solar a considerable boost. The technology is also obviously well suited to off-grid generation and thus to areas without well developed infrastructure.</p><p>47/53 0a3c5a2395ed61be11492a7dbf31b8fe.doc 2018/1/9</p><p>Both photovoltaic and concentrated solar thermal technologies have clear room for improvement. It is not unreasonable to imagine that in a decade or two new technologies could lower the cost per watt for photovoltaics by a factor of ten, something that is almost unimaginable for any other non-carbon electricity source.</p><p>Disadvantages: The ultimate limitation on solar power is darkness. Solar cells do not generate electricity at night, and in places with frequent and extensive cloud cover, generation fluctuates unpredictably during the day. Some concentrated solar thermal systems get around this by storing up heat during the day for use at night (molten salt is one possible storage medium), which is one of the reasons they might be preferred over photovoltaics for large installations. Another possibility is distributed storage, perhaps in the batteries of electric and hybrid cars (see page 810).</p><p>Another problem is that large installations will usually be in deserts, and so the distribution of the electricity generated will pose problems. A 2006 study by the German Aerospace Centre proposed that by 2050 Europe could be importing 100 GW from an assortment of photovoltaic and solar thermal plants across the Middle East and North Africa. But the report also noted that this would require new direct-current high-voltage electricity distribution systems.</p><p>A possible drawback of some advanced photovoltaic cells is that they use rare elements that might be subject to increases in cost and restriction in supply. It is not clear, however, whether any of these elements is either truly constrained — more reserves might be made economically viable if demand were higher — or irreplaceable.</p><p>Verdict: In the middle to long run, the size of the resource and the potential for further technological development make it hard not to see solar power as the most promising carbon-free technology. But without significantly enhanced storage options it cannot solve the problem in its entirety. </p><p>Ocean energy</p><p>J. TAYLOR The oceans offer two sorts of available kinetic energy — that of the tides and that of the waves. Neither currently makes a significant contribution to world electricity generation, but this has not stopped enthusiasts from developing schemes to make use of them. There are undoubtedly some places where, thanks to peculiarities of geography, tides offer a powerful resource. In some situations that potential would best be harnessed by a barrage that creates a reservoir not unlike that of a hydroelectric dam, except that it is refilled regularly by the pull of the Moon and the Sun, rather than being topped up slowly by the runoff of falling rain. But although there are various schemes for tidal barrages under discussion </p><p>48/53 0a3c5a2395ed61be11492a7dbf31b8fe.doc 2018/1/9</p><p>— most notably the Severn Barrage between England and Wales, which proponents claim could offer as much as 8 GW — the plant on the Rance estuary in Brittany, rated at 240 MW, remains the world's largest tidal-power plant more than 40 years after it came into use.</p><p>There are also locations well suited to tidal-stream systems — submerged turbines that spin in the flowing tide like windmills in the air. The 1.2 MW turbine installed this summer in the mouth of Strangford Lough, Northern Ireland, is the largest such system so far installed.</p><p>Most technologies for capturing wave power remain firmly in the testing phase. Individual companies are working through an array of potential designs, including machines that undulate on waves like a snake, bob up and down as water passes over them, or nestle on the coastline to be regularly overtopped by waves that power turbines as the water drains off. The European Marine Energy Centre's test bed off the United Kingdom's Orkney Islands, where manufacturers can hook up prototypes to a marine electricity grid and test how well they withstand the pounding waves, is a leading centre of research. Pelamis Wave Power, a company based in Edinburgh, UK, for instance, has moved from testing there to installing three machines off the coast of Portugal, which together will eventually generate 2.25 MW.</p><p>Costs: Barrage costs differ markedly from site to site, but are broadly comparable to costs for hydropower. At an estimated cost of £15 billion (US$30 billion) or more, the capital costs of the Severn Barrage would be about $4 million per megawatt. A 2006 report from the British Carbon Trust, which spurs investment in non-carbon energy, puts the costs of tidal-stream electricity in the $0.20–0.40 per kilowatt-hour range, with wave systems running up to $0.90 per kilowatt-hour. Neither technology is anywhere close to the large-scale production needed to significantly drive such costs down.</p><p>Capacity: The interaction of Earth's mass with the gravitational fields of the Moon and the Sun is estimated to produce about 3 TW of tidal energy— rather modest for such an astronomical source (although enough to play a key role in keeping the oceans mixed — see Nature 447, 522–524; 2007). Of this, perhaps 1 TW is in shallow enough waters to be easily exploited, and only a small part of that is realistically available. EDF, a French power company developing tidal power off Brittany, says that the tidal-stream potential off France is 80% of that available all round Europe, and yet it is still little more than a gigawatt.</p><p>The power of ocean waves is estimated at more than 100 TW. The European Ocean Energy Association estimates that the accessible global resource is between 1 and 10 terawatts, but sees much less than that as recoverable with current technologies. An analysis in the MRS Bulletin in April 2008 holds that about 2% of the world's coastline has waves with an energy density of 30 kW m−1, which would offer a technical potential of about 500 GW for devices working at 40% efficiency. Thus even with a huge amount of development, wave power would be unlikely to get close to the current installed hydroelectric capacity.</p><p>Advantages: Tides are eminently predictable, and in some places barrages really do offer the potential for large-scale generation that would be significant on a countrywide scale. Barrages also offer some built-in storage potential. Waves are not constant — but they are more reliable than winds.</p><p>ADVERTISEMENT</p><p>49/53 0a3c5a2395ed61be11492a7dbf31b8fe.doc 2018/1/9</p><p>Disadvantages: The available resource varies wildly with geography; not every country has a coastline, and not every coastline has strong tides or tidal streams, or particularly impressive waves. The particularly hot wave sites include Australia's west coast, South Africa, the western coast of North America and western European coastlines. Building turbines that can survive for decades at sea in violent conditions is tough. Barrages have environmental impacts, typically flooding previously intertidal wetlands, and wave systems that flank long stretches of dramatic coastline might be hard for the public to accept. Tides and waves tend by their nature to be found at the far end of electricity grids, so bringing back the energy represents an extra difficulty. Surfers have also been known to object …</p><p>Verdict: Marginal on the global scale.</p><p>See Editorial, page 805. Reported and written by Quirin Schiermeier, Jeff Tollefson, Tony Scully, Alexandra Witze and Oliver Morton.</p><p> References</p><p>1. Key World Energy Statistics 2007 (International Energy Agency, 2007). 2. Hohmeyer, O. & Trittin, T. (eds) Proc. IPCC Scoping Meeting on Renewable Energy Sources 20–25 January 2008, Lübeck, Germany (Intergovernmental Panel on Climate Change, 2008). 3. Smil, V. Energy in Nature and Society: General Energetics of Complex Systems (MIT Press, 2008). 4. Metz, B., Davidson, O., Bosch, P., Dave, R. & Meyer, L. (eds) Climate Change 2007: Mitigation of Climate Change (Cambridge Univ. Press, 2007). </p><p>Comments</p><p>Reader comments are usually moderated after posting. If you find something offensive or inappropriate, you can speed this process by clicking 'Report this comment' (or, if that doesn't work for you, email [email protected]). For more controversial topics, we reserve the right to moderate before comments are published.</p><p> Thanks for much needed information on energy alternatives. Did the authors consider the possibility of harvesting some of the 50,000 billion metric tons of Hydrogen that the Sun discards each year in the solar wind? Until there is better evidence that CO2 causes global warming, it may be premature to report, "So a global response to climate </p><p>50/53 0a3c5a2395ed61be11492a7dbf31b8fe.doc 2018/1/9</p><p> change must involve a move to carbon-free sources of electricity." http://myprofile.cos.com/manuelo09 o Report this comment o 13 Aug, 2008 o Posted by: O M </p><p> Excellent review of energy generation alternatives. Two quick comments. First, the article says that hydropower is "second only to fossil fuels". It says this same thing about biomass. Which is it? Second, in the disadvantages for hydropower, the release of methane and CO2 from decomposing biomass is sited. I have heard this quoted from anti-dam people before and it strikes me as a little deceptive. Are you saying that without the dam, the biomass would *not* decompose? That's a bit like saying that the reason leaves fall off trees is because of nearby dams. Also, it is important to note the CO2 generated by rotting vegetation was only recently pulled from the atmosphere. This is a net zero carbon emission and is part of the normal seasonal cycle of the earth as seen in the Keeling curve. One might even argue that a dam facilitates the removal of carbon from the atmosphere because some of the biomass is captured as sediment which is not released from the bottom of the catchment basin. But that's probably miniscule. o Report this comment o 14 Aug, 2008 o Posted by: David Myer </p><p> Thanks for a great summary of energy generation pros and cons! However, I think the debate on energy technologies misses one critical element: our behaviour as energy users. Clearly, sustainable and carbon-free energy generation is a great goal and some very innovative solutions are appearing. It would be equally impressive to see some of the same enthusiasm directed toward 'energy education', or seeking ways to simply use less. Ultimately, we will need to question the assumption that energy generation capacity must always increase. Better to do it sooner than later. regards o Report this comment o 14 Aug, 2008 o Posted by: adam steer </p><p> David: hydropower is second in terms of electricity generation capacity, while biomass is second overall: most biomass is burned in fires and stoves, though, not used to generate electricity Oliver Morton o Report this comment o 16 Aug, 2008 o Posted by: Oliver Morton </p><p> This is an excellent review but, further to Adam's comment, we tend to concentrate on the supply side and neglect improving energy efficiency which, particularly in the case of housing, can have considerable health and wellbeing impacts. I live in Christchurch, NZ, and at the current price of electricity here (ca. 8p a unit)it is estimated that some 54 000 households out of the 386 000 in the city are affected by 'fuel poverty'(>10% of income is required to achieve adequate warmth). Improvements in insulation etc. would give substantial social and health benefits that increasing power generation would not deliver</p><p>51/53 0a3c5a2395ed61be11492a7dbf31b8fe.doc 2018/1/9</p><p> o Report this comment o 17 Aug, 2008 o Posted by: T F J Taylor </p><p> For David Myer's question: http://www.google.com/search? q=biomass+reservoir+decomposition+anoxic+methane o Report this comment o 18 Aug, 2008 o Posted by: hank roberts </p><p> Not to belabor the point made in other postings on increasing efficiency as a carbon-free strategy for meeting electricity demand, but there is much hard data available on the costs and potential of this approach. Setting higher efficiency standards for appliances, establishing building codes requiring high efficiency lighting, better insulation so that air conditioning costs are lower and use of natural light are all examples of how we can use electricity more efficiently. Harvesting these "negawatts" may be the fastest and cheapest way to cut carbon emissions. Amory Lovins and his Rocky Mountain Institute have spent decades studying this approach. o Report this comment o 19 Aug, 2008 o Posted by: Harry Read </p><p> I was very disapointed that algea farming was not mentioned under bio mass anergy. Algea can produce 20000 gallons of suel oil per acre per year. One tenth the state of New Mexico could provide all of the oil we need. No imported oil and the oil supplied would be carbon neutrol. If you don't believe it look up algea farming on the internet. o Report this comment o 21 Aug, 2008 o Posted by: john clark </p><p> The capacity factor of solar cited is of course quite location sensitive, so it inappropriate to quote a single capacity factor as you do when you write, "Of all renewables, solar currently has the lowest capacity factor, at about 14%." As a counter- example, the capacity factor of the Stirling Energy Systems plant being built in Victorville, CA for Southern California Edison is estimated as 23.9%. The company Ausra claims to have a cost-effective Thermal Energy Storage for their Concentrated Solar Power system. They claim in their whitepaper that they could power the U.S. 24x365. They write, "The 2005/6 U.S. national grid had a generating capacity of 1067 GW and non-coincident peak load of 789 GW. Based on the current technology, a CLFR with SM3 and storage would require 1.5 square miles for 177 MW, translating a national land requirement equal to 23,418 km^2 or a square with 153 km sides." o Report this comment o 29 Aug, 2008 o Posted by: Earl Killian </p><p>52/53 0a3c5a2395ed61be11492a7dbf31b8fe.doc 2018/1/9</p><p> "Algea", nothing. The energy problems of the world will be solved by a website I went to that reduced my computer's electricity consumption to -2 kW. I know I wrote down the URL somewhere. There was frost on the cooling air outlet. I think energy carriers are important (http://www.eagle.ca/~gcowan/235_248.pdf ). o Report this comment o 30 Aug, 2008 o Posted by: Graham Cowan </p><p> The biomass portion of this article neglects the large possibilities from bioengineering of algae and plants to produce easily extracted liquid fuels and biochemical products using tailored biosynthetic pathways. Current genetic engineering techniques now make readily feasible the ideas put forth by Melvin Calvin over 40 years ago. He was just a bit ahead of his time. For example one can readily imagine large expanses of poor quality land being used to produce fuel from algae using sewage as a source of nutrients and salt water as the water source. This would avoid the use of farmland for biofuels with the concommitant effect on food prices we already see happening. o Report this comment o 30 Aug, 2008 o Posted by: Donald Condliffe </p><p> You appear to have neglected solar power satellites - perhaps not feasible immediately, but the obstacles are engineering ones, not theoretical breakthroughs. One of the above technologies (probably nuclear) could provide enough capacity until a workable solar power satellite system could be developed. We don't have to limit ourselves to the sunlight that hits the earth... o Report this comment o 02 Sep, 2008 o Posted by: Alan Barker </p><p> A wellcome overview! Just to mention that a summary of this review has appeared en français at http://www.sauvonsleclimat.typepad.fr/ It contains a couple of critical comments on some of the proposed figures for the future. o Report this comment o 05 Sep, 2008 o Posted by: Jean-Marc Bonneville </p><p> One thing is for sure. Liquid fuels, biofuels or otherwise, are going to become a precious commodity worth their weight in gold. We need to prioritize finding ways to use as little as possible. I'm skeptical we will find ways to produce liquid biofuels without destroying carbon sinks and biodiversity or driving up the cost of food, especially in the short term. And if we ever do, you can bet that producing them will be incredibly expensive compared to simply pumping an energy dense liquid out of a hole in the ground.</p><p>53/53</p>
Details
-
File Typepdf
-
Upload Time-
-
Content LanguagesEnglish
-
Upload UserAnonymous/Not logged-in
-
File Pages53 Page
-
File Size-