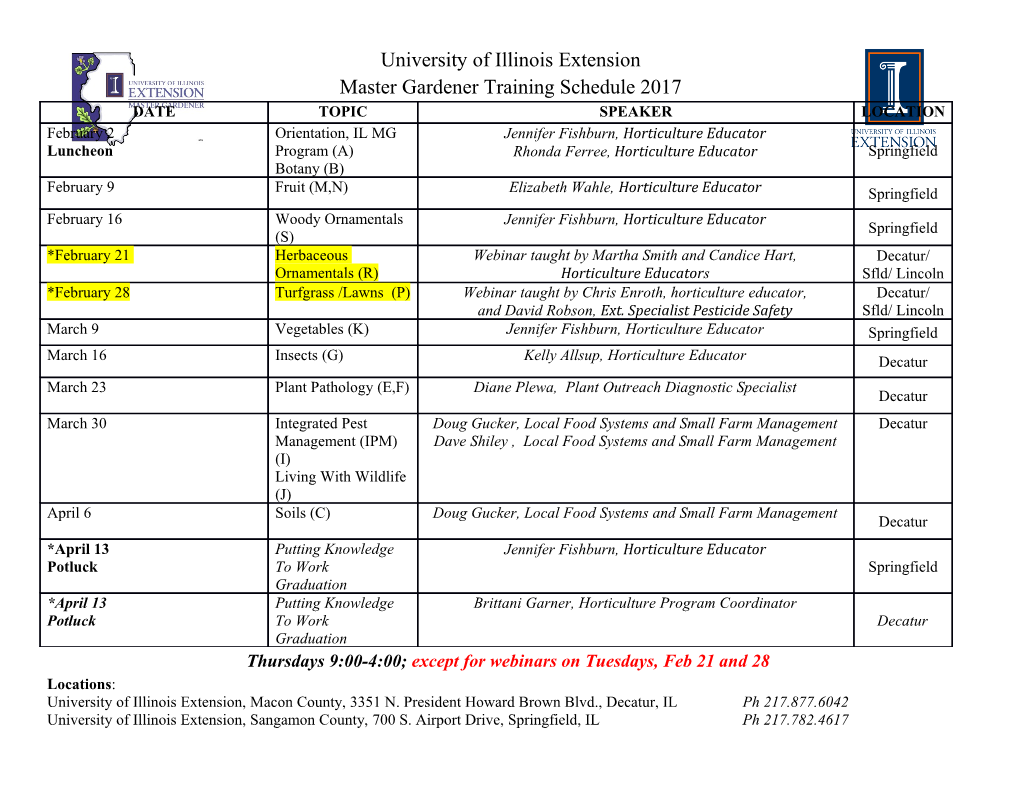
Lithium Methyl Carbonate as a Reaction Product of Metallic Lithium and Dimethyl Carbonate Guorong V. Zhuang*,Z, Hui Yang*, and Philip N. Ross, Jr.* Environmental Energy Technologies Division Lawrence Berkeley National Laboratory, Berkeley, CA 94720, USA Kang Xu* and T. Richard Jow* U. S. Army Research Laboratory, Adelphi, MD 20783, USA Abstracts To improve the understanding of passive film formation on metallic lithium in organic electrolyte, we synthesized and characterized lithium methyl carbonate (LiOCO2CH3), a prototypical component of the film. The chemical structure of this compound was characterized with Nuclear Magnetic Resonance (NMR) and Fourier Transform Infrared Spectroscopy (FTIR), and its thermal stability and decomposition pathway was studied by thermo-gravimetric analysis (TGA). The FTIR spectrum of chemically synthesized compound enabled us to resolve multiple products in the passive film on lithium in dimethyl carbonate (DMC). Lithium methyl carbonate is only one of the components, the others being lithium oxalate and lithium methoxide. ____________________________________________ * Electrochemical Society Active Member ZEmail: [email protected] 1 Introduction In a seminal paper, Aurbach et al.1 proposed that surface films formed on metallic lithium in propylene carbonate (PC) are not composed primarily of lithium carbonate, as 2 3 suggested in earlier work by Bro and Dey , but of lithium alkyl carbonates, ROCO2Li, where R = alkyl group. This conclusion was reached based on ex-situ FTIR analysis of the surface layers, and comparison with spectra for reference compounds taken either from the literature or from compounds synthesized in the course of that study. In addition to PC, some experiments were also described for the linear carbonate, diethylcarbonate (DEC), using the same procedures. It was reported that lithium metal is not passivated in DEC, but dissolves completely to form a dark brown solution. The products were isolated, analyzed by FTIR and reported to be a mixture of lithium ethylcarbonate (CH3CH2OCO2Li) and lithium ethoxide (CH3CH2OLi). This paper was pioneering and formed the basis for later work, especially by Aurbach and co-workers 4,5. However, the actual IR spectra of the lithium alkyl carbonate reference compounds were not published either in reference 1 or in later work, only tabulations of frequencies and relative intensities (s = strong, m = medium, w = weak) were given. Comparison of any experimental spectra with these tabulations is therefore very subjective. In the present work, we present for the first time a complete IR spectrum for an important reference compound, lithium methyl carbonate, and relate this spectrum to that from the surface of metallic lithium immersed from dimethyl carbonate (DMC). The expected chemical reduction path of DMC on metallic Li surface, based on the studies by both Aurbach and co-workers1 and previous work from this laboratory6 is: - 0 2CH3OCO2CH3 + 2e + 2Li → 2LiOCO2CH3 +CH3CH3↑ (1) 2 - 0 • CH3OCO2CH3 + e + Li → CH3OLi + CH3OCO ? (2) The reaction paths are, however, not completely understood, as only the solid products have been analyzed by spectroscopy (XPS or IR) and that product identification has not been unambiguous. It is also unclear whether these two reaction paths are in parallel, occurring simultaneously at different rates, or in series, i.e. methoxide is actually the product of a subsequent reduction of methyl carbonate, as in - 0 2LiOCO2CH3 + 2e + 2Li → LiO2CCO2Li + 2 CH3OLi (3) We also present here data for the thermal decomposition of lithium methyl carbonate, which can be useful in understanding thermal studies of passive films on lithium or carbon anodes in lithium batteries7,8. Experimental Lithium methyl carbonate (LiOCO2CH3) was synthesized at Army Research Laboratory, via a route modified from that used by Aurbach et al.1. The entire process of synthesis and purification was conducted in a dry-room with dew point below -80 oC. Methanol was first dried over 3Ǻ molecular sieve and then over neutral alumina until the moisture level was less than 5 ppm as determined by Karl-Fischer titration (Mettler- Toledo). Lithium hydride (LiH) was then slowly added to the excess amount of dried methanol (CH3OH) through a solid addition funnel at a rate to keep the reactant temperature below 40 oC. At the end of the LiH-addition the reactant was heated to reflux at ca. 80 oC for an hour. During the process additional amount of methanol was added to ensure that a clear methanolic solution of lithium methoxide (LiOCH3) was obtained after cooling down to room temperature. Analytic grade carbon dioxide (CO2) was introduced into the solution before passing through two gas bubblers containing concentrated 3 sulfuric acid (H2SO4). The reaction of LiOCH3 with CO2 is obviously exothermic and white crystal flakes was observed to precipitate. The CO2-injection was continued for several hours to ensure the completion of the LiOCH3 conversion, and the excess methanol was then evaporated. The obtained crystal was washed three times by the dried methanol to remove any possible remnants of unreacted LiOCH3. The final product was vacuum dried at room temperature. 1 The identification of LiOCO2CH3 as the synthesis product was conducted via H- and 13C-NMR (Oxford 400 MHz NMR spectrometer) at University of Maryland. All deutered solvents used in NMR characterization were dried by 3 Å molecular sieves. When trace amount of LiOCO2CH3 was dissolved in deutered dimethyl sulfoxide 1 (DMSO-d6), the solution showed H-signal at 3.27 ppm (singlet, for C(2)H(1)3-O(3)-) as 13 well as C-signals at 51 ppm (singlet, for C(2)H(1)3-O(3)-) and 157 ppm (singlet, for – C(1)O(3)-). Due to the limited solubility of LiOCO2CH3 in DMSO-d6, the observed carbonyl NMR signal was very weak. To confirm the presence of sp2-hybridized – O(1)C(1)O(2)- structure and to avoid interference from the DMSO-d6 signals, we deliberately decomposed the LiOCO2CH3 in D2O, and carried out further NMR measurements. The 1H-signal of decomposition mixture was shifted to 3.39 ppm, while a 13 conspicuous C peak was detected at 160 ppm, indicating that CH3OD and lithium carbonate (Li2CO3) were formed as a result of hydrolysis. Therefore, the synthesized crystalline product was confirmed to be LiOCO2CH3. A lithium (Li) foil (99.999%, 1.5 mm thick) and dimethyl carbonate (DMC) (Aldrich, anhydrous 99%+, H2O < 20 ppm) were used for studying the surface chemical reaction of Li and DMC. The experiments were conducted in Ar-filled glove box, with O2 4 content < 1 ppm and H2O content < 2 ppm. The surface reaction was initiated by scraping off the native surface layer on metallic Li foil while in DMC solution, followed by exposure of the fresh surface to DMC for one minute. After removing the Li foil from the DMC solution, the excess DMC was allowed to evaporate from the sample surface in the glovebox prior to transferring to the FTIR spectrometer. FTIR analysis of all solid samples, including the solid remnants from LiOCO2CH3 after TGA experiment and the surface film on metallic Li, were conducted with the attenuated total internal reflection (ATR) method described in previous publications9,10. The FTIR measurements were performed in a He environment, and under no circumstances were the sample exposed to air. All the spectra were acquired at a resolution of 4 cm-1 and summed over 512 scans. A Thermo-gravimetric Analyzer (TGA model 2960, TA Instruments) and a Fourier Transform Infrared (FTIR) Spectrometer (Nexus 470, Nicolet) equipped with a temperature controlled transmission gas cell were used for thermal analysis by dynamic TGA-FTIR. Thermal analysis by TGA-FTIR measures the change in sample weight as a function of temperature at a heating rate of 10 K·min-1 while simultaneously analyzing the composition of volatile components evolved from sample as a result of thermal decomposition. The gas phase products were eluted to the FTIR gas cell by Ar carrier gas at a flow rate of 220 ml•min-1. The FTIR spectra were continuously acquired at a resolution of 4 cm-1 and summed over 32 scans during the thermo-gravimetric (TG) measurements. The TGA and FTIR are coupled via quartz capillary array. During the analysis, both FTIR gas cell and capillary array were maintained at 200 oC to prevent gas absorption or condensation on gas cell and capillary walls. To ensure an inert atmosphere 5 during the thermal analysis, the TGA was housed in argon (Ar) atmosphere glovebag. The optical path was sufficiently purged that the infrared signal from residual moisture and CO2 was much smaller than that from the reaction products. The inert atmosphere working condition of the TGA-FTIR apparatus was checked using thermal analysis copper oxalate following work of Mullens et al.11. All the samples were transferred from an Ar atmosphere glovebox to the TGA sample chamber using a portable vacuum carrier with no transient air exposure. The mass loss measurements reported in this work have an accuracy of ±1%. Results and Discussion The purity of the synthesized lithium methyl carbonate is confirmed by the FTIR spectrum in Fig.1, which is absent of any form of crystallized water, lithium hydroxide (LiOH) or absorbed water as indicated by the featureless spectral region above 3000 cm-1. The amount of lithium carbonate (Li2CO3) in the sample is negligible as well. The molecular structure of lithium methyl carbonate was obtained from an ab-initio quantum chemical calculation at the HF/6-31G(d,p) level12 for the two conformations shown in Fig.1. The systematic error in calculated vibrational frequencies at the HF level was corrected by using empirical scaling factor of 0.892913. Calculated vibrational frequencies based on the converged geometries are tabulated in Table 1. Our calculated vibrational frequencies are very similar to those reported earlier for the same molecular conformations14 using essentially the same computational methodology.
Details
-
File Typepdf
-
Upload Time-
-
Content LanguagesEnglish
-
Upload UserAnonymous/Not logged-in
-
File Pages24 Page
-
File Size-