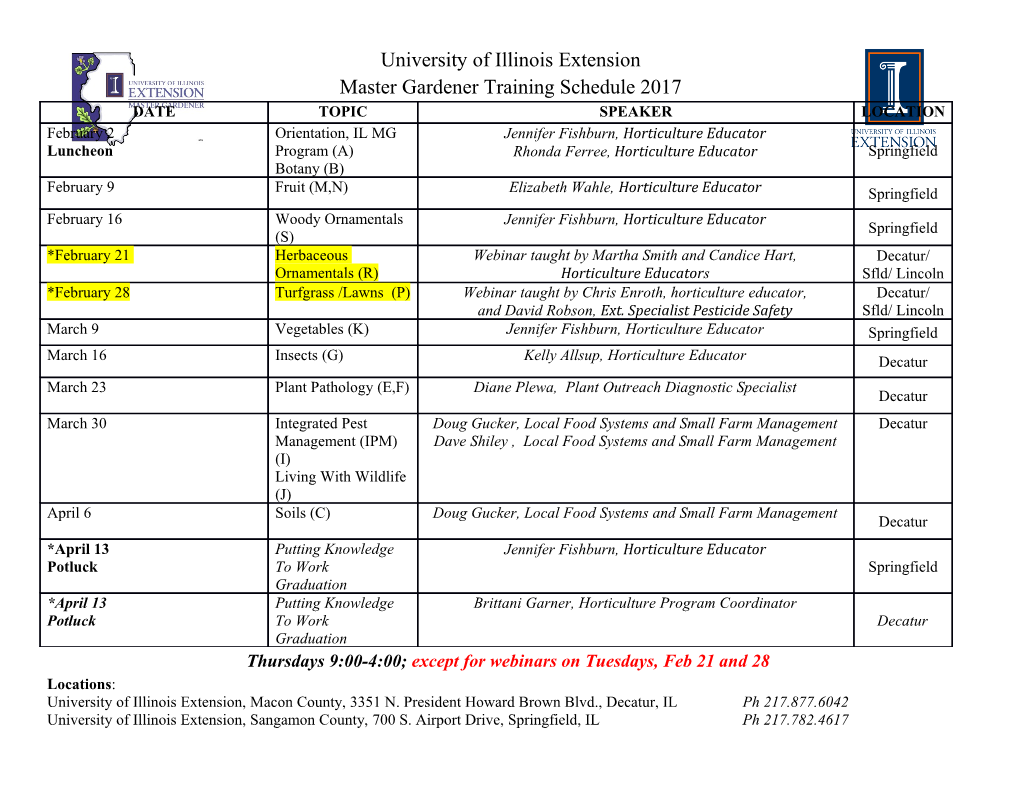
1 Growth and form of the mound in Gale Crater, Mars: Slope-wind 2 enhanced erosion and transport 3 Edwin S. Kite1, Kevin W. Lewis2, Michael P. Lamb1, Claire E. Newman,3 and Mark I. 4 Richardson3 5 6 1Geological and Planetary Sciences, California Institute of Technology, MC 150-21, Pasadena CA 7 91125, USA. 8 2Department of Geosciences, Princeton University, Guyot Hall, Princeton NJ 08544, USA. 9 3Ashima Research, Pasadena CA 91125, USA. 10 11 ABSTRACT 12 Ancient sediments provide archives of climate and habitability on Mars. Gale Crater, the landing 13 site for the Mars Science Laboratory (MSL), hosts a 5 km high sedimentary mound (Mt. Sharp / 14 Aeolis Mons). Hypotheses for mound formation include evaporitic, lacustrine, fluviodeltaic, and 15 aeolian processes, but the origin and original extent of Gale’s mound is unknown. Here we show 16 new measurements of sedimentary strata within the mound that indicate ~3° outward dips oriented 17 radially away from the mound center, inconsistent with the first three hypotheses. Moreover, 18 although mounds are widely considered to be erosional remnants of a once crater-filling unit, we 19 find that the Gale mound’s current form is close to its maximal extent. Instead we propose that the 20 mound’s structure, stratigraphy, and current shape can be explained by growth in place near the 21 center of the crater mediated by wind-topography feedbacks. Our model shows how sediment can 22 initially accrete near the crater center far from crater-wall katabatic winds, until the increasing 23 relief of the resulting mound generates mound-flank slope-winds strong enough to erode the 1 24 mound. The slope-wind enhanced erosion and transport (SWEET) hypothesis indicates mound 25 formation dominantly by aeolian deposition with limited organic carbon preservation potential, and 26 a relatively limited role for lacustrine and fluvial activity. Morphodynamic feedbacks between 27 wind and topography are widely applicable to a range of sedimentary and ice mounds across the 28 Martian surface, and possibly other planets. 29 30 INTRODUCTION 31 Most of Mars’ known sedimentary rocks are in the form of intra-crater or canyon mounded 32 deposits like the mound in Gale crater (Hynek et al., 2003), but identifying the physical 33 mechanism(s) that explain mound growth and form has proved challenging, in part because these 34 deposits have no clear analog on Earth. The current prevailing view on the formation of intra-crater 35 mounds is that sedimentary layers (i.e., beds) completely filled each crater at least to the summit of 36 the present-day mound (Malin and Edgett, 2000). Subsequent aeolian erosion, decoupled from the 37 deposition of the layers, is invoked to explain the present-day topography (Andrews-Hanna et al., 38 2010; Murchie et al., 2009). Evaporitic, lacustrine, fluviodeltaic, and aeolian processes have each 39 been invoked to form the layers (e.g., Anderson and Bell, 2010; Andrews-Hanna et al., 2010; ; 40 Irwin et al., 2005; Niles and Michalski, 2009; Pelkey et al., 2004; Thomson et al, 2011). If the 41 sedimentary rocks formed as subhorizontal layers in an evaporitic playa-like setting, then >>106 42 km3 must have been removed to produce the modern moats and mounds (Zabrusky et al., 2012). 43 These scenarios predict near-horizontal or slightly radially-inward dipping layers controlled by 44 surface or ground water levels. 45 46 GALE MOUND LAYER ORIENTATIONS 2 47 To test this, we obtained bed-orientation measurements from six one-meter-scale stereo elevation 48 models using planar fits to extracted bedding profiles via the technique of Lewis and Aharonson 49 (2006). Each elevation model is constructed from a High Resolution Imaging Science Experiment 50 (HiRISE) stereopair using the method of Kirk et al. (2008). Individual measurements were rejected 51 where the angular regression error was greater than 2°, and the 81 remaining measurements were 52 averaged for each site to reduce uncertainty further, with the results shown in Figure 1. We find 53 that layers have shallow but significant dips away from the mound center, implying 3-4 km of pre- 54 erosional stratigraphic relief if these dips are extrapolated to the rim. Measurements of the marker 55 bed of Milliken et al. (2010) show that its elevation varies by >1km, confirming that beds are not 56 planar. Postdepositional radially-outward tilting is unlikely. Differential compaction of porous 57 sediments, flexural response to the mound load, or flexural response to excavation of material from 58 the moat would tilt layers inward, not outward. Layers targeted by MSL near the base of Gale’s 59 mound show no evidence for halotectonics or karstic depressions at kilometer scale, and 60 deformation by mantle rebound would require the Gale mound to accumulate extremely quickly 61 (Figure 2).Therefore, these measurements permit only a minor role for deposition mechanisms that 62 preferentially fill topographic lows (e.g., playa, fluviodeltaic or lacustrine sedimentation), but are 63 consistent with aeolian processes (Figure 2). This suggests the mound grew with its modern shape, 64 and that the processes sculpting the modern mound may have molded the growing mound. 65 66 SLOPE WIND EROSION ON MARS 67 Mars is a windy place; saltating sand-sized particles are in active motion on Mars, at rates that 68 predict aeolian erosion of bedrock at 10-50 µm/yr (Bridges et al., 2012). Aeolian erosion of rock 69 has occurred within the last ~1-10 Ka (Golombek et al., 2010) and is probably ongoing. Because of 70 Mars’ thin atmosphere, slope winds are expected to dominate the circulation in craters and canyons 3 71 (Spiga and Forget, 2009). We have performed mesoscale (~4km horizontal resolution) simulations 72 of Gale Crater using the MarsWRF general circulation model (Richardson et al., 2007; Toigo et al., 73 2012) with embedded high-resolution nests, and these provide further evidence that winds in Gale 74 are expected to peak on the steep crater wall and mound slopes. Downslope-oriented yardangs, 75 crater statistics, exposed layers, and lag deposits suggest that sedimentary mounds in Valles 76 Marineris (e.g. Murchie et al., 2009) and Gale are being actively eroded by slope winds. Slope- 77 enhanced winds appear to define both the large-scale and small-scale topography and stratigraphy 78 of the polar layered deposits (e.g. Holt et al., 2010; Smith and Holt, 2010), and radar sounding of 79 intracrater ice mounds near the north polar ice sheet proves that these grew from a central core, 80 suggesting a role for slope winds (Conway et al., 2012). Most of the ancient stratigraphy explored 81 by the Opportunity rover is aeolian (Metz et al., 2009), and aeolian deposits likely represent a 82 volumetrically significant component of the sedimentary rock record, including within the strata of 83 the Gale mound (Anderson and Bell, 2010). Evidence for fluvial reworking within sedimentary 84 mounds is comparatively limited and/or localized (e.g., Thomson et al., 2011; Irwin et al., 2005). 85 Quasi-periodic bedding at many locations including the upper portion of Gale’s mound implies 86 slow (~30 µm/yr) orbitally-paced accumulation (Lewis et al., 2008). These rates are comparable to 87 the modern gross atmospherically-transported sediment deposition rate (101-2 µm/yr ; Drube et al., 88 2010), suggesting that aeolian processes may be responsible for the layers. Sedimentary strata 89 within Valles Marineris are meters-to-decameters thick, laterally continuous, have horizontal-to- 90 draping layer orientations, and display very few angular unconformities (Fueten et al., 2010). 91 These data suggest that sedimentary deposits formed by the accretion of atmospherically- 92 transported sediment (ash, dust, impact ejecta, ice nuclei, or rapidly-saltating sand) formed readily 93 on early Mars as well as in the more recent past (Grotzinger et al., 2010; Cadieux et al., 2011). 4 94 Slope-wind erosion of indurated or lithified aeolian deposits cannot explain the outward 95 dips observed at Gale unless the topographic depression surrounding the mound (i.e., the moat) 96 seen in Figure 1 was present throughout mound growth. This implies a coupling between mound 97 primary layer orientations, slope winds, and mound relief. 98 99 MODEL 100 To explore this feedback, we aimed to develop the simplest possible model that can account for the 101 structure and stratigraphy of Mars' equatorial sedimentary rock mounds. In one horizontal 102 dimension (x), topographic change dz/dt is given by 103 104 dz/dt = D – E (1) 105 106 where D is an atmospheric source term and E(x,t) is erosion or sediment entrainment rate. Initial 107 model topography (Figure 3) is a basalt (nonerodible) crater/canyon with a flat floor of half-width 108 R and 20° slopes. Although dipping beds in the mound suggest a dominant role for aeolian 109 processes in mound growth, our model does not preclude the possibility of intermittent 110 fluvial/lacustrine deposition, which may have been partly later reworked by aeolian processes. To 111 highlight the role of slope winds in building mounds through erosion and deposition, we initially 112 assume D is constant and uniform (e.g., Niles and Michalski, 2009; Fergason and Christensen, 113 2008; Holt et al., 2010) and focus on E as the driver of wind entrainment and erosion. E typically 114 has a power-law dependence on maximum shear velocity magnitude at the air-sediment interface, 115 U: 116 E = k U α (2) 5 117 where k is an erodibility factor that depends on substrate grainsize and induration/cementation, and 118 α ~ 3-4 for sand transport, soil erosion, and rock abrasion (Kok et al., 2012). We assume that 119 sediments have some cohesive strength, most likely due to processes requiring liquid water (e.g., 120 damp or cemented sediment, bedrock, crust formation).
Details
-
File Typepdf
-
Upload Time-
-
Content LanguagesEnglish
-
Upload UserAnonymous/Not logged-in
-
File Pages29 Page
-
File Size-