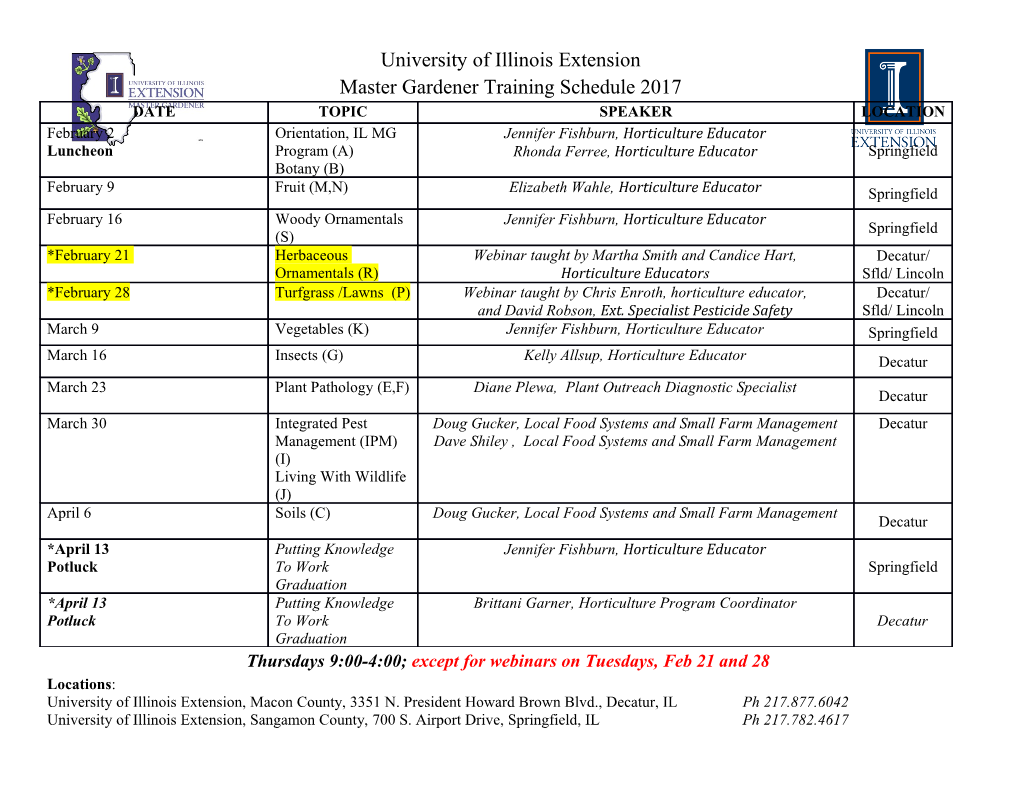
A Small Symmetric BIBDs and Abelian Difference Sets We provide a summary of known existence and nonexistence results for “small” symmetric BIBDs and Abelian difference sets. In Table A.1, we list all parameter triples (v, k, λ) in which λ(v − 1)=k(k − 1), v/2 ≥ k ≥ 3, and 3 ≤ k ≤ 15 (if k > v/2, then apply block complementation, which was presented as Theorem 1.32, and/or Exercise 3.1). We use the following abbreviations in Table A.1. • “Singer” denotes a Singer difference set (Theorem 3.28). • “QR” denotes a quadratic residue difference set (Theorem 3.21). • “H” denotes a (4m − 1, 2m − 1, m − 1)-BIBDconstructed from a Hadamard matrix of order 4m via Theorem 4.5. • ( ) “PGd q ” denotes a projective geometry (Theorem 2.14). • “BRC” denotes the Bruck-Ryser-Chowla Theorems (Theorems 2.16 and 2.19). • “MT” denotes the Multiplier Theorem (Theorem 3.33). For existence of certain symmetric BIBDs and for the nonexistence of cer- tain difference sets, we refer to external sources. Note also that existence of a difference set implies the existence of the corresponding symmetric BIBD, and nonexistence of a symmetric BIBD implies nonexistence of a difference set with the same parameters in any (Abelian or non-Abelian) group. kvλ SBIBD notes difference set notes ( ) 371yes PG2 2 yes Singer ( ) 4131 yes PG2 3 yes Singer ( ) 5211 yes PG2 4 yes Singer 5 11 2 yes H yes QR ( ) 6311 yes PG2 5 yes Singer 6162 yes yes Example3.4 7431 no BRC no 7222 no BRC no ( ) 7153 yes PG3 2 ,H yes Singer ( ) 8571 yes PG2 7 yes Singer 8292 no BRC no ( ) 9731 yes PG2 8 yes Singer 9372 yes yes Example3.24 9 25 3 yes [113, Table 5.25] no [10, Table A.3.1] 9 19 4 yes H yes QR ( ) 10 91 1 yes PG2 9 yes Singer 10 46 2 no BRC no 10 31 3 yes no MT, p = 7 11 111 1 no [74] no MT, p = 2, 5 11 56 2 yes [113, Table 5.25] no [10, Table A.3.1] 11 23 5 yes H yes QR ( ) 12 133 1 yes PG2 11 yes Singer 12 67 2 no BRC no 12 45 3 yes yes Example 3.5 12 34 4 no BRC no 13 157 1 unknown no Example 3.38 13 79 2 yes [113, Table 5.25] no MT, p = 11 13 53 3 no BRC no ( ) 13 40 4 yes PG3 3 yes Singer 13 27 6 yes H yes QR ( ) 14 183 1 yes PG2 13 yes Singer 14 92 2 no BRC no 15 211 1 no BRC no 15 106 2 no BRC no 15 71 3 yes [113, Table 5.25] no [10, Table A.3.1] 15 43 5 no BRC no 15 36 6 yes yes Example 3.6 ( ) 15 31 7 yes PG5 2 ,H yes Singer, QR Table A.1. Small Symmetric BIBDs and Abelian Difference Sets B Finite Fields In this appendix, we give a brief summary of basic facts concerning finite fields. We provide definitions of the main concepts, several illustrative ex- amples, and statements of some important theorems, but no proofs. A reader wanting to study finite fields in more detail can consult a suitable algebra textbook. Definition B.1. A finite field is a triple (X, ×, +) such that X is a finite set with |X|≥2 and “×”and“+” are binary operations on X such that the following conditions are satisfied: 1. addition is closed; i.e., for any a, b ∈ X, a + b ∈ X; 2. addition is commutative; i.e., for any a, b ∈ X, a + b = b + a; 3. addition is associative; i.e., for any a, b, c ∈ X, (a + b)+c = a +(b + c); 4. 0 is an additive identity; i.e., for any a ∈ X, a + 0 = 0 + a = a; 5. for any a ∈ X, there exists an additive inverse of a, denoted −a, such that a +(−a)=(−a)+a = 0; 6. multiplication is closed; i.e., for any a, b ∈ X, a × b ∈ X; 7. multiplication is commutative; i.e., for any a, b ∈ X, a × b = b × a; 8. multiplication is associative; i.e., for any a, b, c ∈ X, (a × b) × c = a × (b × c); 9. 1 is a multiplicative identity; i.e., for any a ∈ X, a × 1 = 1 × a = a; − 10. for any a ∈ X\{0},thereexistsamultiplicative inverse of a, denoted a 1, − − such that a × a 1 = a 1 × a = 1; 11. the distributive property is satisfied; i.e., for any a, b, c ∈ X, (a + b) × c = (a × c)+(b × c),anda× (b + c)=(a × b)+(a × c). The order of the finite field (X, ×, +) is the integer |X|. Suppose that (X, ×, +) is a finite field. Properties 1–5 establish that (X, +) is an Abelian group, and properties 6–10 show that (X\{0}, ×) is an Abelian group. Here are some familiar examples of fields. Example B.2. (R, ×, +) and (Q, ×, +) are both (infinite) fields. 282 B Finite Fields Example B.3. If p is prime, then every nonzero element of Zp has a multiplica- tive inverse, and (Zp, ×, +) is a finite field of order p. A finite ring is a triple (X, ×, +) that satisfies every property of a finite field except for property 10. Example B.4. If m ≥ 2 is an integer, then (Zm, ×, +) is a finite ring. If m is composite, then it is easy to see that (Zm, ×, +) is not a field as follows. Sup- pose that d is a divisor of m,where1 < d < n.Thend does not have a multiplicative inverse modulo m, so property 10 is violated. There exist finite fields that are not of prime order. In fact, there is a finite field with q elements whenever q = pn, p is prime, and n ≥ 1 is an integer. We will now describe very briefly how to construct such a field when n > 1. First, we need several definitions. Definition B.5. Suppose p is prime. Define Zp[x] to be the set of all polynomials in the indeterminate x in which the coefficients are elements of Zp.((Zp[x], ×, +) is a ring, where multiplication and addition of polynomials are defined in the usual way except that all coefficients are reduced modulo p.) 1. For f (x), g(x) ∈ Zp[x], we say that f (x) divides g(x) (notation: f (x) | g(x)) if there exists q(x) ∈ Zp[x] such that g(x)=q(x) f (x). 2. For f (x) ∈ Zp[x],definedeg( f ),thedegree of f , to be the highest exponent in a term of f . 3. Suppose f (x), g(x), h(x) ∈ Zp[x],anddeg( f )=n ≥ 1.Wedefine g(x) ≡ h(x)(mod f (x)) if f (x) | (g(x) − h(x)). Notice the resemblance of the definition of congruence of polynomials to that of congruence of integers. We are now going to define a finite ring of polynomials “modulo f (x)”, which we denote by Zp[x]/( f (x)). The construction of Zp[x]/( f (x)) from Zp[x] is based on the idea of congruences modulo f (x) and is analogous to the construction of Zm from Z. Suppose deg( f )=n. If we divide g(x) by f (x),weobtaina(unique) quotient q(x) and remainder r(x),where g(x)=q(x) f (x)+r(x) and deg(r) < n. B Finite Fields 283 This can be done by the usual long division of polynomials. It follows that any polynomial in Zp[x] is congruent modulo f (x) to a unique polynomial of degree at most n − 1. n Now we define the elements of Zp[x]/( f (x)) to be the p polynomials in Zp[x] of degree at most n − 1. Addition and multiplication in Zp[x]/( f (x)) are defined as in Zp[x], followed by a reduction modulo f (x). Equipped with these operations, Zp[x]/( f (x)) is a finite ring. Recall that Zm is a field if and only if m is prime. A similar situation holds for Zp[x]/( f (x)). The analog of primality for polynomials is irreducibility, which we define as follows. Definition B.6. A polynomial f (x) ∈ Zp[x] is said to be an irreducible polyno- ( ) ( ) ∈ Z [ ] mial if there do not exist polynomials f1 x , f2 x p x such that ( )= ( ) ( ) f x f1 x f2 x , ( ) > ( ) > where deg f1 0 and deg f2 0. Irreducible polynomials of all possible orders exist. More precisely, we have the following theorem. Theorem B.7. For any prime p and for any integer n ≥ 1, there exists an irre- ducible polynomial f (x) ∈ Zp[x] having degree n. The relevance of irreducible polynomials to the construction of finite fields is as follows. Theorem B.8. Suppose p is prime and f (x) ∈ Zp[x].ThenZp[x]/( f (x)) is a (finite) field if and only if f (x) is irreducible. Here is an example to illustrate the concepts described above. Example B.9. Let’s construct a finite field having eight elements. This can be Z [ ] done by finding an irreducible polynomial of degree three in 2 x .Itissuf- ficient to consider the polynomials having constant term equal to 1 since any polynomial with constant term 0 is divisible by x and hence is reducible. There are four such polynomials: ( )= 3 + f1 x x 1 ( )= 3 + + f2 x x x 1 ( )= 3 + 2 + f3 x x x 1 ( )= 3 + 2 + + f4 x x x x 1.
Details
-
File Typepdf
-
Upload Time-
-
Content LanguagesEnglish
-
Upload UserAnonymous/Not logged-in
-
File Pages21 Page
-
File Size-