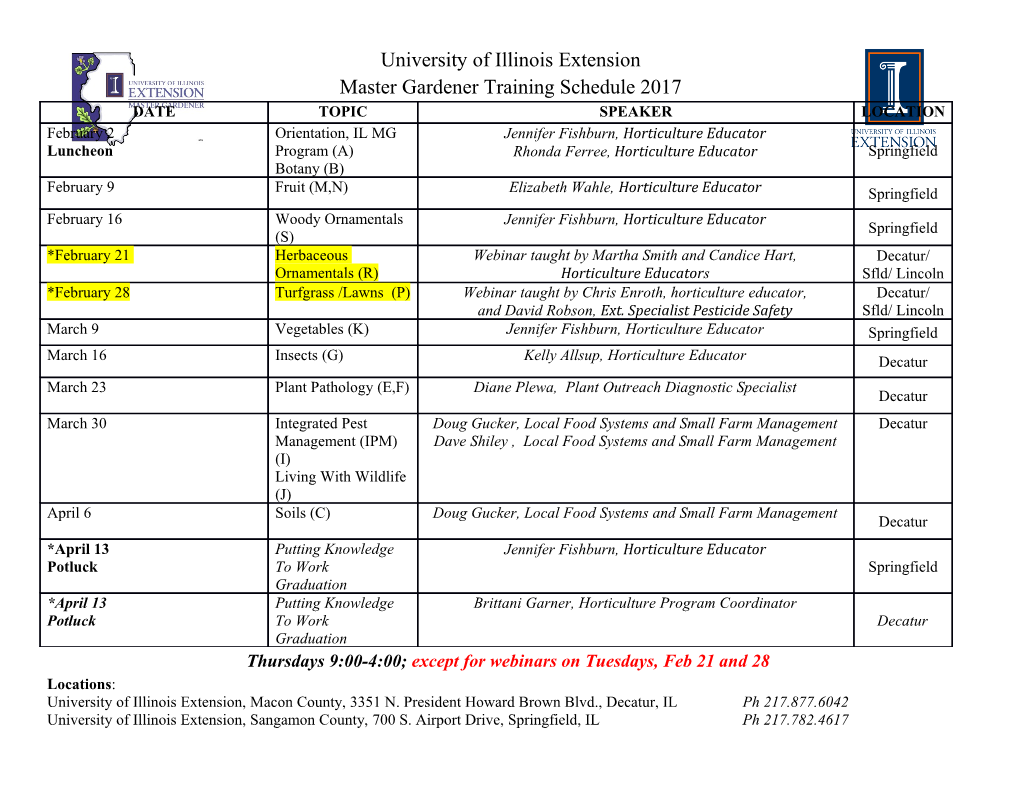
1 Revised version to Biogeosciences 2 Methane related changes in prokaryotes along geochemical profiles in sediments 3 of Lake Kinneret (Israel) 4 I. Bar Or1, E. Ben-Dov2,3, A. Kushmaro*2,5,6, W. Eckert4 and O. Sivan1 5 6 1Department of Geological and Environmental Sciences, Ben-Gurion University of the Negev, 7 Be'er- Sheva, P.O. Box 653, 8410501, Israel. 8 2Avram and Stella Goldstein-Goren Department of Biotechnology Engineering, Ben-Gurion 9 University of the Negev, Be'er- Sheva, P.O. Box 653, 8410501, Israel. 10 3Department of Life Sciences, Achva Academic College, Achva, M.P. Shikmim 79800, Israel. 11 4Israel Oceanographic and Limnological Research, The Yigal Allon Kinneret Limnological 12 Laboratory, PO Box 447, 14950 Migdal, Israel. 13 5National Institute for Biotechnology in the Negev, Ben-Gurion University of the Negev, P.O. Box 14 653, Be'er-Sheva 8410501, Israel 15 6School of Materials Science and Engineering, Nanyang Technological University, Singapore 16 17 * Corresponding author: Mailing address: Department of Biotechnology Engineering, Ben-Gurion 18 University of the Negev, P.O. Box 653, Be’er-Sheva 8410501, Israel. Phone: 972-8-6479.024. Fax: 19 972-8-6472.983. E-mail: [email protected]. 20 21 Keywords: anaerobic methane oxidation, iron reduction, archaeal classification, bacterial 22 classification, Lake Kinneret. 23 24 Abstract. Microbial methane oxidation is the primary control on the emission of the greenhouse 25 gas methane into the atmosphere. In terrestrial environments, aerobic methanotrophic bacteria are 26 largely responsible for this process. In marine sediments a coupling of anaerobic oxidation of 27 methane (AOM) with sulfate reduction, often carried out by a consortium of anaerobic 28 methanotrophic archaea (ANME) and sulfate reducing bacteria, consumes almost all methane 29 produced within marine sediments. Motivated by recent evidence for AOM with iron(III) in Lake 30 Kinneret sediments, the goal of the present study was to link the geochemical gradients in the lake 31 porewater to the microbial community structure. Screening of archaeal 16S rRNA gene sequences 32 revealed a shift from hydrogenotrophic to acetoclastic methanogens with depth. The observed 33 changes in microbial community structure suggest possible direct and indirect mechanisms for the 1 34 AOM coupled to iron reduction in deep sediments. Members of the Nitrospirales order increased 35 with depth, suggesting their involvement in iron reduction together with Geobacter genus and 36 "reverse methanogenesis". An indirect mechanism through sulfate and ANMEs seems less probable 37 due to the absence of ANME sequences. This is despite the abundant sequences related to sulfate 38 reducing bacteria (Deltaproteobacteria) together with the occurrence of dsrAB in the deep sediment 39 that could indicate the production of sulfate (disproportionation) from S0 for sulfate-driven AOM. 40 The presence of the functional gene pmoA in the deep anoxic sediment together with sequences 41 related to Methylococcales suggests the existence of a second unexpected indirect pathway - aerobic 42 methane oxidation pathway in an anaerobic environment. 43 2 44 1. Introduction 45 Chemical profiles in the porewater of aquatic sediments reflect the sequence of microbially 46 mediated redox reactions that are driven by the availability of both, electron donors and of suitable 47 electron acceptors. The latter are depleted in the order of decreasing chemical potential, beginning 48 with oxygen and proceeding through nitrate, manganese and iron oxides, and then sulfate. Below 49 the main zone of sulfate reduction, the fermentation of organic carbon leads to the formation of 50 methane (CH4) by the process of methanogenesis (Froelich et al., 1979). 51 The produced methane is isotopically depleted in 13C, with values of ~−50 to −110‰ (Schoell, 52 1988), and the residual dissolved inorganic carbon (DIC) pool is enriched by an isotopic 53 fractionation factor (ε) of 50 to 70‰ (e.g., (Borowski et al., 2000; Whiticar, 1999)). When the 54 produced methane diffuses into a zone with a suitable electron acceptor, it can be consumed by 55 microbial oxidation (methanotrophy), the main process by which the important greenhouse methane 56 is prevented from escaping into the atmosphere. While in the terrestrial environment, aerobic 57 methanotrophy is the dominant process (Chistoserdova et al., 2005), in anaerobic marine sediments 58 archaea are found to consume the majority of upward diffusing methane coupled to sulfate 59 reduction (Knittel and Boetius, 2009; Thauer, 2010; Valentine, 2002). 60 Evidence from lipids and from fluorescence in situ hybridization (FISH) showed that a 61 consortium of archaea and sulfate reducing bacteria are involved in this anaerobic methane 62 oxidation (AOM) (Boetius et al., 2000; Hinrichs et al., 1999; Orphan et al., 2001). To date, three 63 groups of anaerobic methanotrophic archaea (ANME), named ANME-1, ANME-2, and ANME-3, 64 are known to perform sulfate driven AOM (Niemann et al., 2006; Orphan et al., 2002). Recently 65 Milucka et al. (2012) demonstrated AOM mediated solely by archaea, where the archaea was shown 66 to oxidize the methane and reduce the sulfate to elemental sulfur. Disproportionating bacteria, also 67 involved in this mechanism, oxidize and reduce this elemental sulfur to sulfate and sulfide, 68 respectively. The carbon isotopic fractionation factor (ε) for this methanotrophic process was shown 69 to be in the range of 4-30‰ (Kinnaman et al., 2007; Whiticar, 1999). Enrichment cultures of 70 ANME from different environments showed a carbon isotopic fractionation of 12-39‰ (Holler et 71 al., 2009). 72 Other electron acceptors were recently shown to drive AOM. Nitrite driven AOM by oxygenic 73 bacteria was observed in two different freshwater ecosystems in Netherlands (Ettwig et al., 2009; 74 Raghoebarsing et al., 2006) and also in peatlands (Zhu et al., 2012). Beal et al. (Beal et al., 2009) 75 showed the potential of manganese and iron-driven AOM in marine sediments, and Egger et al., 76 (2014) showed it in brackish costal sediments. In our recent study (Sivan et al., 2011), we provided 77 in situ geochemical evidence for AOM coupled to microbial iron reduction below the main 3 78 methanogenesis zone in Lake Kinneret (LK) sediments, where dissolved sulfate and nitrate are 79 absent. However, the mechanism that is responsible for this process was not investigated. The goal 80 of the present study is to explore the possible microbial communities that may be involved in the 81 iron driven AOM in LK sediments. This is accomplished by combining chemical and isotope 82 analyses of porewater samples along a depth profile from LK sediments with molecular biological 83 techniques. By using specific functional genes related to dissimilatory sulfate reductase (dsr) for 84 sulfate reducers (Klein et al., 2001), methyl-coenzyme M reductase (mcr) for methanogens and 85 anaerobic methanotrophs (Hallam et al., 2003) and particulate methane monooxygenase (pmmo) for 86 aerobic methanotrophs (McDonald et al., 2008) we pinpoint the most likely candidate for this 87 relatively unknown process. 88 1.1. Study site 89 Located in northern Israel, Lake Kinneret (LK, Fig.1) is a warm monomictic subtropical lake. 90 Typical concentrations of major electron acceptors in the water column during the mixed period are 91 35–50 μM nitrate and 600 μM sulfate (Adler et al., 2011; Nishri et al., 2000; Serruya et al., 1974). 92 In the spring, the newly formed epilimnion is characterized by increasing temperatures and 93 enhanced phytoplankton development, while in the hypolimnion heterotrophic microorganisms 94 gradually deplete oxygen and then nitrate. Organic matter degradation by bacterial iron and 95 manganese reduction takes place below the thermocline in the summer, and in the end of the 96 stratification period, sulfate reduction starts in the bottom water. In the upper part of the sediment, 97 sulfate reduction is the dominant microbial process year round, and below depth of 5 cm this 98 process is mostly replaced by methanogenesis (Adler et al., 2011; Eckert and Conrad, 2007). Total 99 iron content (Fe(tot)) in the sediment increases with depth till 18 cm and then remains uniform 100 around 550 µmol/g Dry Weight (Eckert, 2000). Mn(II) concentration is about 13 µmol/g Dry 101 Weight in the sediment (Serruya, 1971). The total carbon (Ctot) in the solid phase shows a decrease 102 from 14 µmol/g Dry Weight in the top part of the sediment to 8 µmol/g Dry Weight in the deep part 103 of the sediment (~30 cm) (Eckert, 2000). 104 105 2. Material and Methods 106 2.1. Sampling 107 Several sediment cores were collected from the center of the lake (Station A, Fig. 1) at a water 108 depth of ~42 m (maximum lake water column depth) at different times using Perspex tubes, 109 measuring 55 cm long by 5 cm in diameter, with a gravity corer. The cores were stored in the dark 110 at 4°C until they were sliced (on the same day or the day after). Core sampling for the microbial 4 111 community study took place in December 2009. Over a dozen of porewater chemical and isotope 112 profiles were conducted seasonally (every 3-4 months) from 2007 to 2013. The slight seasonal 113 changes allowed using typical geochemical profiles (Adler et al., 2011) in order to sample for the 114 microbiology communities in the different electron acceptors zones and to correlate between the 115 microbial sampling and the geochemical profiles. 116 2.2. Geochemical analyses 117 Cores were divided into 2-cm slices from top to bottom under a constant flow of N2 using a 118 slicing device. About 1.5 ml of each sediment slice was transferred into N2-flushed crimp bottles 13 119 containing 5 ml of 1.5 N NaOH for the headspace measurements of CH4 and δ CCH4 (after Sivan et 120 al., 2011).
Details
-
File Typepdf
-
Upload Time-
-
Content LanguagesEnglish
-
Upload UserAnonymous/Not logged-in
-
File Pages51 Page
-
File Size-