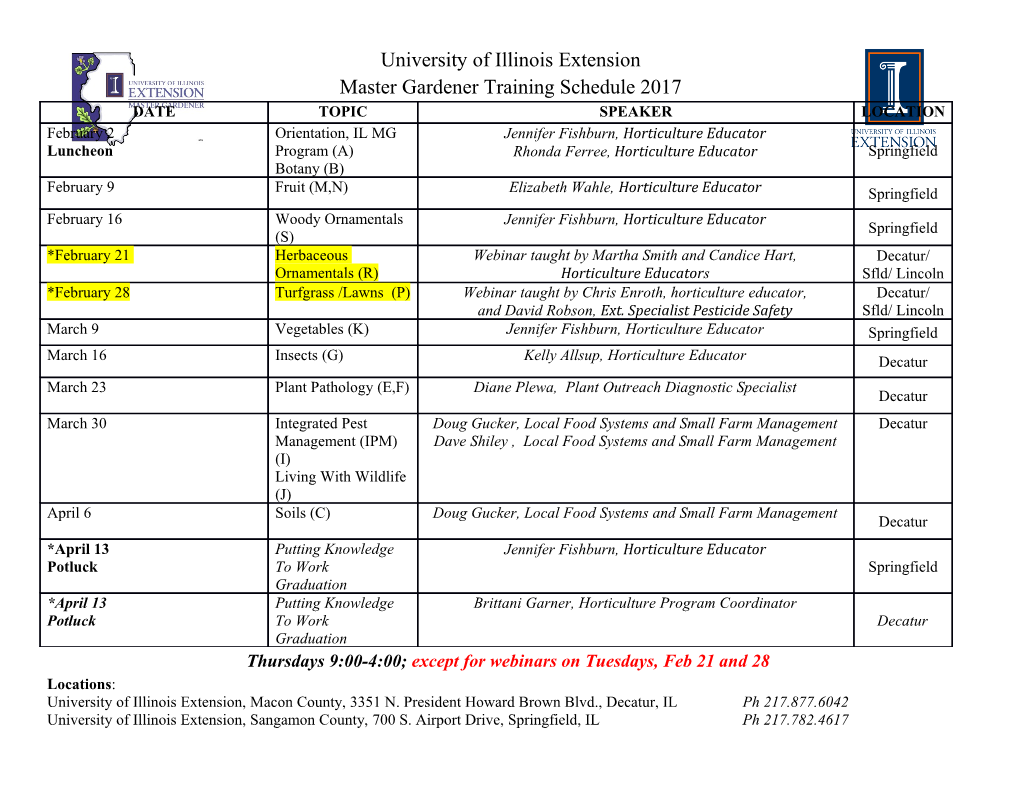
Astronomy Instrument Principles: Constraints from photon mean free path Karsten Kretschmer (MPE-Gamma) 1. Effects of the Earth‘s atmosphere 2. Transparency of the universe 3. Detection principles 4. Astronomical instruments: specific methods and problems The Earth’s Atmosphere (I) n Infrared Radio- Light l X-Rays Waves Radiation a Gamma-Rays UV Radiatio Optic O 100km , N, 3 O o t s due nd a 50km B n o i rpt on o i Abs flect e 10km R CO2,H2O,O3... log10ν log 10λ Fig. 1 Observations from the ground are only possible in certain frequency ranges, caused by the variation of atmospheric transparency with frequency. The Earth’s Atmosphere (II) The radio window: ≈ 3 MHz – 3 THz, ≈ 100 m – 100 µm • Lower limit: plasma frequency of the ionosphere: 2 Nee ω p = ε0me Waves with lower frequency are reflected by the ionospheric plasma. • Upper limit: absorption by atmospheric molecules, mainly H2O, CO2 for wavelengths less than about 1 mm. Fig. 2 The Earth’s Atmosphere (III) The optical window: ≈ 1.5 µm – 300 nm, ≈ 0.8 eV – 4 eV • Lower limit: molecular absorption lines (H2O, CO2) • Upper limit: molecular absorption (O3, O2, N2) For higher energies (E > 100 eV), the dominant absorption comes from photoelectric absorption, moving to Compton scattering and pair production for E > 1 MeV and E > 10 MeV, respectively. Fig. 3 Transparency of the Universe (I) The universe is in general not transparent to electromagnetic radiation • Radio: interstellar plasma: varying electron density → varying index of refraction → “interstellar scintillation” • Infrared-optical-ultraviolet: interstellar dust causes frequency dependent absorption high-frequency radiation is absorbed faster → “reddening” Fig. 4 Fig. 5 Transparency of the Universe (II) Fig. 6 Transparency of the Universe (III) • X-Rays: interstellar absorption (photoelectric effect) decreases with rising photon energy. For E larger than a few keV it is unimportant. τ ∝ ω −8/3 ⋅ N dl ()h ∫ H • For photons in the high gamma-ray energies, electron-positron pair production with background photons becomes important. The energy threshold for pair production with a photon from the cosmic microwave background is on the order of 100 TeV. Fig. 7 Detection Principles • Two general categories of detection can be distinguished: coherent and incoherent • Coherent: measurement of the EM field amplitude hν << kT: wavelike effects dominate used for radio and submillimeter receivers (less than ≈ 100 GHz) • Incoherent: measurement of the EM field intensity - Bolometers: detect temperature increase - energy gap devices: The energy of the incoming photon is used to lift particles over a potential barrier. Instruments: Radio • Angular resolution limited by diffraction (λ/D) → large diameters, interferometry • Background: Galaxy, atmosphere, telescope and receiver • Collectors: metallic reflectors, wire antennas for long wavelengths • Energy resolution: electronic filters Fig. 8 Instruments: Infrared • Angular resolution limited by diffraction for far- and mid-IR, by atmospheric turbulence (“seeing”) in the near-IR •Intense background from thermal emission of the telescope, atmosphere, dust in the solar system and in the galaxy, detector noise → cooling, chopping • Detectors: small band- gap semiconductors, doped semiconductors • Collectors: metallic mirrors, metal-coated glass mirrors, lenses • Energy resolution: filters, grating spectrographs Fig. 9 Instruments: Optical • Angular resolution limited by atmospheric turbulence (“seeing”) • Background: scattered light in the atmosphere, detector noise • Detectors: semiconductors (mainly Si), photographic emulsions, human eye • Collectors: metal-coated glass mirrors, lenses • Energy resolution: filters, prism or grating spectrographs • Single photon detection becomes possible: Photomultipliers, semiconductor devices (APD), energy resolution possible with superconducting tunnel junctions Instruments: Ultraviolet and X-Ray •For λ < 120 nm, similar to optical • For shorter wavelengths, normal incidence mirrors no longer reflect, but absorb the light → grazing incidence mirrors required, practical for E < 10 keV • Detectors: semiconductors, gas ionisation counters, microchannel plates • Background: charged particles (cosmic rays, solar flares, radiation belts) Fig. 10 Instruments: Gamma-Ray • Focusing only possible in special cases → use of collimators/coded apertures for E < 1 MeV use of particle physics methods for E > 1 MeV: tracking of individual particles • Detectors: semiconductors, scintillators, … • Background: charged particles (cosmic rays, solar flares, radiation belts), radioactivity in the telescope • Photons with E > 100 GeV generate electromagnetic showers in the atmosphere, detectable through their Čerenkov radiation. Fig. 11 Neutrinos and gravitational waves • Neutrino astronomy: early state, only two known sources (sun, SN1987A) • Detectors: - radiochemical: inverse beta decay, no angular resolution, time resolution ≈ weeks - real-time: ν interaction creates energetic charged particle → scintillation, Čerenkov - need for big detectors! • Gravitational waves: no direct detection yet • Detectors: - resonators: measurement of mechanical oscillations in a test mass - interferometers: measurement of light propagation time variations Fig. 12 Resources and References • Longair, Malcolm S.; High energy astrophysics; Cambridge UP Figures 3, 4, 7, 9 and 11 were taken from this book, fig. 1 adapted. It gives a good introduction to the processes of radiation production and detection. While mainly oriented towards high energies, it is not limited to this area. • Fig. 2 is from H. Vogel, Gerthsen Physik, fig. 5 from “dtv-Atlas Astronomie” • Figures 6, 8, 10 and 12 were taken from the respective web sites: http://www.eso.org/outreach/press-rel/pr-2001/pr-01-01.html http://www.mpifr-bonn.mpg.de/div/effelsberg/index_e.html http://wave.xray.mpe.mpg.de/rosat http://www-sk.icrr.u-tokyo.ac.jp/sk/.
Details
-
File Typepdf
-
Upload Time-
-
Content LanguagesEnglish
-
Upload UserAnonymous/Not logged-in
-
File Pages15 Page
-
File Size-