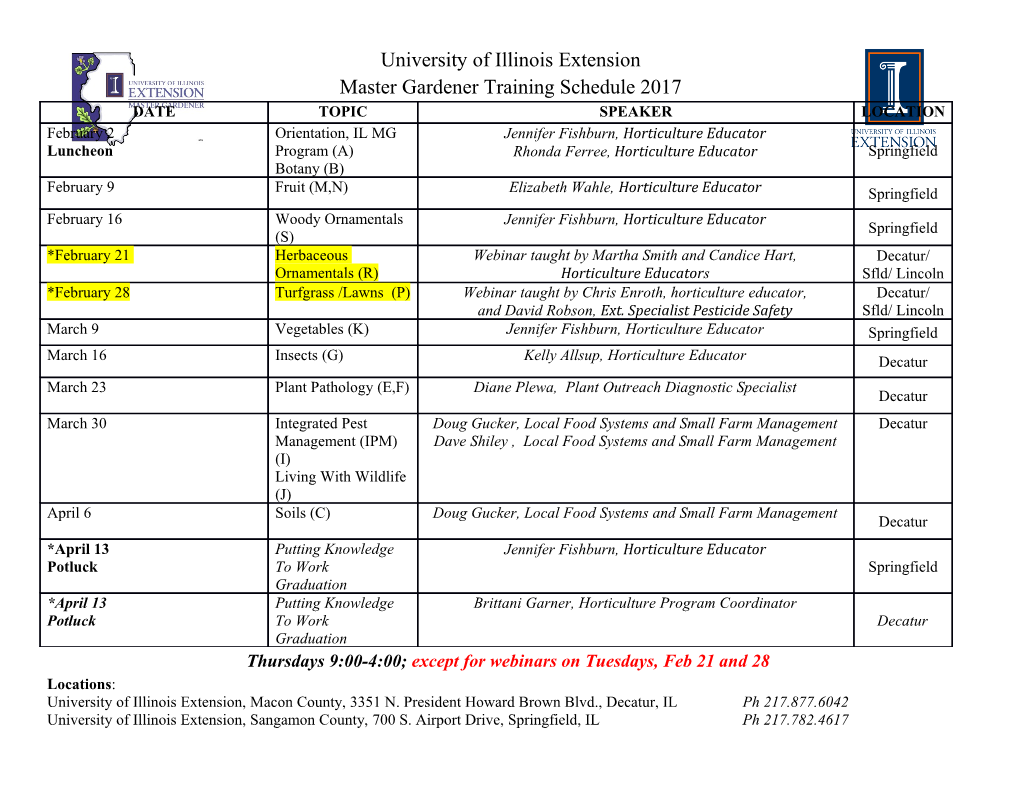
RIEMANNIAN GEOMETRY: SOME EXAMPLES, INCLUDING MAP PROJECTIONS SVANTE JANSON Abstract. Some standard formulas are collected on curvature in Rie- mannian geometry, using coordinates. (There are, as far as I know, no new results). Many examples are given, in particular for manifolds with constant curvature, including many well-known map projections of the sphere and several well-known representations of hyperbolic space, but also some lesser known. 1. Introduction We collect general formulas on curvature in Riemannian geometry and give some examples, with emphasis on manifolds with constant curvature, in particular some standard map projections of the sphere (Section 6) and some standard representations of hyperbolic space (Section 7). We work with coordinates, and therefore represent tensors as indexed quantities Ai1;:::;ip with arbitrary numbers p 0 and q 0 of indices (con- j1;:::;jq > > travariant and covariant, respectively); we say that this tensor is of type (p; q), or a (p; q)-tensor. Some formulas and calculations are much more elegant in coordinate-free formulations, see e.g. [4], but our emphasis is on explicit calculations. We assume knowledge of basic tensor calculus, including covariant and contravariant tensors, contractions and raising and lowering of indices. 1.1. Basic notation. We consider an n-dimensional Riemannian manifold M and a coordinate system (x1; : : : ; xn), i.e. a diffeomorphic map of an open n subset of M onto an open subset of R ; we assume n > 2. All indices thus i take the values 1; : : : ; n. We use the Einstein summation convention: a i Pn i means i=1 a i. ij The metric tensor is gij, and the inverse matrix is denoted g : ij −1 g = gij (1.1) or in the usual notation with coordinates ik i g gkj = δj; (1.2) i where δj denotes the Kronecker delta. (We may also write δij when more convenient, which often is the case when considering an example with a given Date: 1 March, 2015; revised 14 March, 2015. 1 2 SVANTE JANSON coordinate system.) Recall that the metric tensor is symmetric: gij = gji and note that contracting the metric tensor or δ yields the dimension: ij i g gij = δi = n: (1.3) We denote the determinant of a 2-tensor A = (Aij) by jAj. In particular, jgj := det(gij): (1.4) It is easily seen, using (1.2) and expansions of the determinant, that @jgj = jgjgij: (1.5) @gij The invariant measure µ on M is given by 1=2 dµ = jgj dx1 ··· dxn: (1.6) (This measure can be defined in an invariant way as the n-dimensional Hausdorff measure on M.) The volume scale is thus jgj−1=2. 1.2. Distortion. The condition number { > 1 of the metric tensor gij (at a given point) is the ratio between the largest and smallest eigenvalue of gij. Thus { = 1 if and only if gij is conformal. It is seen, by taking an ON basis diagonalizing gij, that the map maps infinitesimally small spheres to 1=2 ellipsoids with the ratio of largest and smallest axes { , and conversely, infinitesimally small spheres on the map correspond to ellipsoids with this ratio of largest and smallest axes. We define p " := 1 − 1={: (1.7) Thus 0 6 " < 1, and " = 0 if and only if gij is conformal. If n = 2, then " is the eccentricity of the ellipses that correspond to (infinitesimally) small circles by either the coordinate map or its inverse. If n = 2, then the two eigenvalues of the matrix gij are, with τ := Tr(gij) = g11 + g22, τ ± pτ 2 − 4jgj g + g ± p(g + g )2 − 4jgj = 11 22 11 22 (1.8) 2 2 and thus g + g + p(g + g )2 − 4jgj g + g + p(g − g )2 + 4g2 = 11 22 11 22 = 11 22 11 22 12 { p 2 p 2 2 g11 + g22 − (g11 + g22) − 4jgj g11 + g22 − (g11 − g22) + 4g12 (1.9) and, by (1.7), 2p(g + g )2 − 4jgj 2p(g − g )2 + 4g2 "2 = 11 22 = 11 22 12 : p 2 p 2 2 g11 + g22 + (g11 + g22) − 4jgj g11 + g22 + (g11 − g22) + 4g12 (1.10) RIEMANNIAN GEOMETRY: SOME EXAMPLES 3 1.3. Derivatives. We denote partial derivatives simply by indices after a comma: pq::: @ pq::: Akl:::;i := Akl::: (1.11) @xi and similarly for higher derivatives. The Levi-Civita connection has the components m mk Γij = g Γkij (1.12) where 1 Γkij = 2 gki;j + gkj;i − gij;k : (1.13) k (Γkij is often denote [ij; k]. Γkij and Γij are also called the Christoffel k symbols of the first and second kind.) Note that Γij is symmetric in i and j: k k Γij = Γji: (1.14) (This says that the Levi-Civita connection that is used in a Riemannian manifold is torsion-free.) The covariant derivative of a tensor field is denoted by indices after a semicolon. Recall that for a function (scalar) f, the covariant derivative equals the usual partial derivative in (1.11): f;i = f;i: (1.15) For a contravariant vector field Ak we have k k k j A;i = A;i + ΓjiA (1.16) and for a covariant vector field Ak we have j Ak;i = Ak;i − ΓkiAj (1.17) and for tensor fields of higher order we similarly add to the partial derivative one term as in (1.16) for each contravariant index and subtract one term as in (1.17) for each covariant index, for example i i i p p i p i p i Ajkl;m = Ajkl;m + ΓpmAjkl − ΓjmApkl − ΓkmAjpl − ΓlmAjkp: (1.18) Recall that the Levi-Civita connection has the property that the covariant derivative of the metric tensor vanishes: gij;k = 0; (1.19) indeed, this together with (1.14) is easily seen to be equivalent to (1.13). Higher covariant derivatives are defined by iteration, for example k f;ij := (f;i);j = f;ij − Γijf;k: (1.20) Recall that the covariant derivative of a tensor field is a tensor field, i.e., invariant in the right way under changes of coordinates, while the partial derivative is not (except for a scalar field, where the two derivatives are the same, see (1.15)). A simple calculation, using integration by parts in coordinate neighbour- hoods together with (1.6), (1.5), (1.16) and (1.19), shows that if f is a 4 SVANTE JANSON smooth function and Ai is a vector field, and at least one of them has com- pact support, then Z Z i i f;iA dµ = − fA;i dµ. (1.21) M M If Aij and Bij are symmetric covariant 2-tensors, we define their Kulkarni{ Nomizu product to be the covariant 4-tensor Aij Bkl := AikBjl + AjlBik − AilBjk − AjkBil: (1.22) We may also use the notation (A B)ijkl. (The notation Aij Bkl is slightly improper, but will be convenient. The notation (A B)ijkl is formally better.) Note that A B = B A. Remark 1.1. The Kulkarni{Nomizu product in (1.22) is the bilinear ex- tension of the map (AiCj;BkDl) 7! AiCj BkDl = (Ai ^ Bj)(Ck ^ Dl) (1.23) where Ai ^ Bj := AiBj − AjBi is the exterior product. 1.4. The Laplace{Beltrami operator. The Laplace{Beltrami operator ∆ is a second-order differential operator defined on (smooth) functions on M as the contraction of the second covariant derivative: ij ij ij k ∆F := g F;ij = g F;ij − g ΓijF;k: (1.24) A function F is harmonic if ∆F = 0. For an extension to differential forms, see e.g. [19, Chapter 6]. The integration by parts formula (1.21) implies that if F and G are smooth functions and at least one of them has compact support, then Z Z Z ij F ∆G dµ = − g F;iG;j dµ = G∆F dµ. (1.25) M M M In particular, if M is compact, then this holds for all smooth F and G and shows that −∆ is a non-negative operator. (For this reason, the Laplace{ Beltrami operator is sometimes defined with the opposite sign.) See further [19]. 1.5. Curves. Let γ :(a; b) ! M be a smooth curve, i.e., a smooth map of an open interval into the Riemannian manifold M. Let Dt denote covariant pq::: differentiation along γ. For a tensor field Akl::: it can be defined by pq::: pq::: i DtAkl::: (γ(t)) = Akl:::;i(γ(t))γ _ (t); (1.26) for example, for a tensor of type (1,1), cf. (1.16){(1.18), p p p j j p i DtAk(γ(t)) = Ak;i + ΓjiAk − ΓkiAj γ_ (t) d = Ap(γ(t)) + Γp Aj γ_ i − Γj Apγ_ i: (1.27) dt k ji k ki j However, this depends only on the values of the tensor field along the curve, pq::: and thus it can be defined for any tensor Akl::: (t) defined along the curve. RIEMANNIAN GEOMETRY: SOME EXAMPLES 5 For each t 2 (a; b), Dtγ(t) =γ _ (t) is the tangent vector at γ(t) with i d i componentsγ _ (t) = dt γ (t); this is called the velocity vector, and the velocity is its length 1=2 i j1=2 kγ_ k := hγ;_ γ_ i = gijγ_ γ_ : (1.28) 2 The acceleration is the vector Dt γ(t) which has components, cf. (1.26){ (1.27), 2 i i i i j k Dt γ = Dtγ_ =γ ¨ + Γjkγ_ γ_ ; (1.29) i i where Γjk = Γjk(γ). Since the covariate derivative Dg = 0 for the Levi-Civita connection, see (1.19), i j i j i j 2 Dt gijγ_ γ_ = gijDtγ_ γ_ + gijγ_ Dtγ_ = 2hDtγ;_ γ_ i = 2hDt γ; γ_ i: (1.30) i j Consequently, γ has constant velocity, i.e., hγ;_ γ_ i = gijγ_ γ_ is constant, if and only if the acceleration is orthogonal to the velocity vector.
Details
-
File Typepdf
-
Upload Time-
-
Content LanguagesEnglish
-
Upload UserAnonymous/Not logged-in
-
File Pages122 Page
-
File Size-