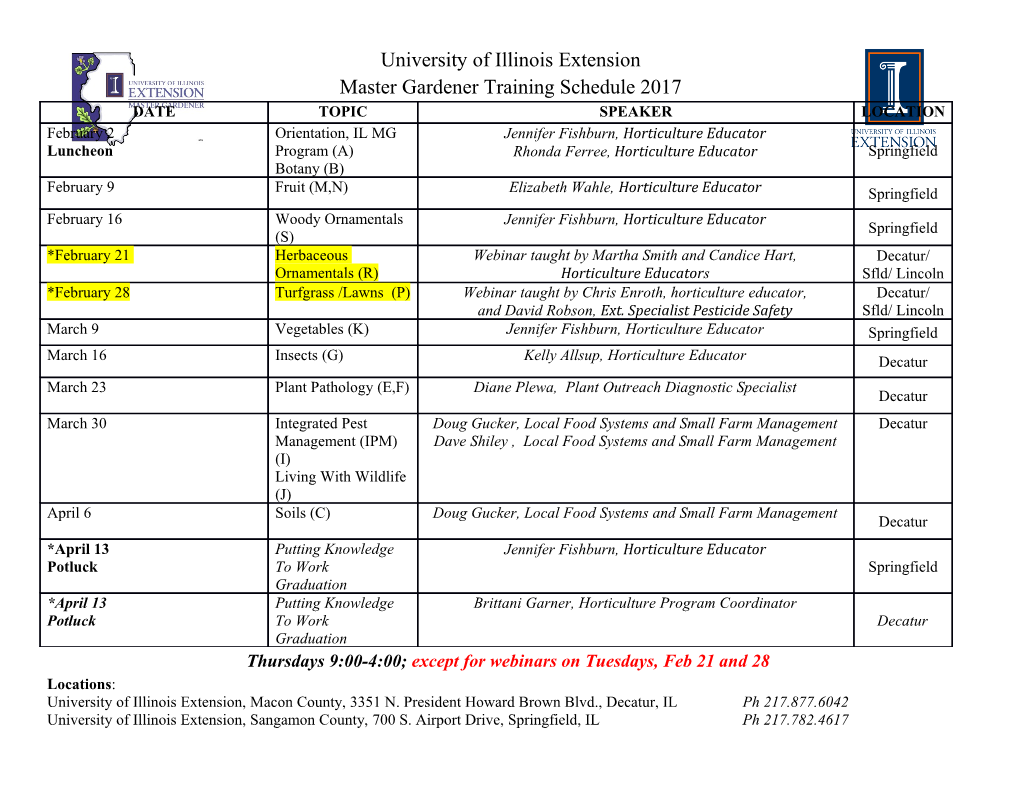
ARTICLES PUBLISHED ONLINE: 29 JUNE 2016 | DOI: 10.1038/NGEO2743 Composition and structure of the shallow subsurface of Ceres revealed by crater morphology Michael T. Bland1*, Carol A. Raymond2, Paul M. Schenk3, Roger R. Fu4, Thomas Kneissl5, Jan Hendrik Pasckert6, Harry Hiesinger6, Frank Preusker7, Ryan S. Park2, Simone Marchi8, Scott D. King9, Julie C. Castillo-Rogez2 and Christopher T. Russell10 Before NASA’s Dawn mission, the dwarf planet Ceres was widely believed to contain a substantial ice-rich layer below its rocky surface. The existence of such a layer has significant implications for Ceres’s formation, evolution, and astrobiological potential. Ceres is warmer than icy worlds in the outer Solar System and, if its shallow subsurface is ice-rich, large impact craters are expected to be erased by viscous flow on short geologic timescales. Here we use digital terrain models derived from Dawn Framing Camera images to show that most of Ceres’s largest craters are several kilometres deep, and are therefore inconsistent with the existence of an ice-rich subsurface. We further show from numerical simulations that the absence of viscous relaxation over billion-year timescales implies a subsurface viscosity that is at least one thousand times greater than that of pure water ice. We conclude that Ceres’s shallow subsurface is no more than 30% to 40% ice by volume, with a mixture of rock, salts and/or clathrates accounting for the other 60% to 70%. However, several anomalously shallow craters are consistent with limited viscous relaxation and may indicate spatial variations in subsurface ice content. lthough Ceres' surface is silicate-rich1–3, its low density undergo viscous relaxation on short timescales13. The arrival of (2,162 kg m−3 (ref.4) from NASA's Dawn mission 5) the Dawn mission5 at Ceres has shown the surface to generally combined with measurements of its shape (that is, the be heavily cratered, including numerous craters with diameter A 6 difference between its equatorial and polar radius, a–c) previously D ∼ 100 km (ref. 14; Supplementary Fig. 1). The mere existence suggested that the dwarf planet is differentiated with a rocky core of these craters, many of which are at low latitudes (that is, warm overlain by a relatively pure-ice layer 50–100-km thick7. In this surface temperatures), belies predictions of a topographically model the rocky surface is probably a lag deposit representing `smooth', ice-rich Ceres13. Here we compare the depths of these the top of a thin (∼1–10 km), mixed ice–rock layer that avoided largest craters with numerical simulations of viscous relaxation to complete melting during differentiation8, although such a layer constrain the physical composition of Ceres' outermost layer. may be unstable to disruption by impacts and Rayleigh–Taylor overturn7,9. Alternatively, Ceres' interior has been modelled Measurement of crater depths on Ceres 10 as an ice-free, porous chondritic assemblage , although the We measured the apparent depths (da) of Ceres' largest, clearly thermal stability of such a model is uncertain11. Dawn's Visible identifiable craters (see ref. 15) using a digital terrain model (DTM) and Infrared Spectrometer (VIR) has not detected widespread derived from Dawn Framing Camera (FC) images with a resolution occurrences of water ice on Ceres' surface2, where it is not of 140 m pixel−1 (from Dawn's High Altitude Mapping Orbit) using thermodynamically stable12. The ice content of the deeper interior stereo-photogrammetry (SPG) methods16. The DTM has a mean remains unknown, and has significant implications for Ceres' vertical error of 12 m and a horizontal resolution similar to the formation and subsequent evolution. An ice-rich subsurface image resolution (140 m). Because viscous relaxation is wavelength would have played a dominant role in shaping Ceres' surface dependent, large craters provide the most stringent constraints morphology. Ceres is warm when compared to the icy worlds on the viscosity required to maintain crater topography (in an of the outer Solar System (for example, ∼50–60 K warmer than infinite half space, large craters relax fastest). We therefore focus Europa), with diurnally averaged equatorial (latitudes <30◦) on craters with diameters between 75 km and 280 km, and for well- temperatures of 150–160 K, mid-latitude (∼50◦) temperatures preserved craters we consider our inventory complete to diameters of ∼140 K, and polar (∼70◦) temperatures of ∼120 K or less12. above 80 km (n D 25 craters, Supplementary Fig. 1). Apparent At such warm surface temperatures, ice is not strong enough to depth (that is, depth relative to the surrounding terrain) rather retain large-scale topography, and impact craters are expected to than the rim depth (dr) is used because the surrounding terrain 1US Geological Survey, Astrogeology Science Center, Flagsta, Arizona 86001, USA. 2Jet Propulsion Laboratory, California Institute of Technology, Pasadena, California 91109, USA. 3Lunar and Planetary Institute, Houston, Texas 77058, USA. 4Columbia University, New York, New York 10027, USA. 5Institute of Geological Sciences, Freie Universität Berlin, Berlin 12249, Germany. 6Institut für Planetologie, Westfälische Wilhelms-Universität, Münster 48149, Germany. 7German Aerospace Center (DLR), Berlin 12489, Germany. 8Southwest Research Institute, Boulder, Colorado 80302, USA. 9Virginia Institute of Technology, Blacksburg, Virginia 24061, USA. 10Department of Earth and Space Sciences, University of California, Los Angeles, California 90095, USA. *e-mail: [email protected] 538 NATURE GEOSCIENCE | VOL 9 | JULY 2016 | www.nature.com/naturegeoscience © 2016 Macmillan Publishers Limited. All rights reserved NATURE GEOSCIENCE DOI: 10.1038/NGEO2743 ARTICLES 70 8 60 6 50 Tethys pure ice 40 Expected depths 4 Lunar Latitude (°) 30 Apparent depth (km) Apparent 20 2 Ganymede 10 100 km 0 0 50 100 150 200 250 300 0123456789 Crater diameter (km) Apparent depth (km) Figure 1 | The apparent depths of Ceres’ largest craters. Black dots Figure 2 | Comparison of the observed depths of Ceres’ largest craters indicate the characteristic depth of each crater. Vertical bars indicate the with the depths expected for an ice-rich subsurface composition as a azimuthal variability of the depth due to variations in the elevation of the function of latitude. Simulations assume a 100-km-diameter crater. Line surrounding terrain (see Methods). Curves for fresh craters on colour corresponds to dierent combinations of ice grain size and crater Tethys (green)17, Ganymede (purple)17 and the Moon (black)18 are shown age: 1 mm, 1 Gyr (purple); 1 mm, 100 Myr (blue); 10 mm, 1 Gyr (orange); and for comparison. The curves have been adjusted to account for crater rims. 10 mm, 100 Myr (red). Symbol size shows crater diameter relative to 100 km, and the horizontal black lines associated with each circle indicate provides the zero-potential surface to which the crater will relax (for azimuthal variations in crater depth. example, a fully relaxed crater can have da D 0, but dr 6D 0). Crater depths were measured by comparing the elevation of the floor to ago and 100 Myr ago, and grain sizes of 1 mm and 10 mm (ref. 20). a characteristic elevation for the surrounding terrain (see Methods Except for Kerwan, Yalode and Urvara (D D 280, 260 and 170 km), and Supplementary Information). the craters included in this investigation are within 40% of the Our measured crater depths are shown in Fig.1. Ceres' diameter of the simulated crater. The uncertainty introduced by topography is highly irregular over short spatial scales. The elevation the use of a generic crater diameter in our simulations is not of terrain surrounding each crater can therefore differ substantially significant compared to the poorly constrained grain size and crater in one azimuthal direction compared to another (see Supplementary formation time. Methods). This variability is encapsulated in the vertical bars Because of the strong temperature dependence of ice rheology associated with each point in Fig.1. These bars should not be (for example, ref. 21), viscous relaxation is expected to produce construed as formal or informal error bars. Rather each point distinct latitudinal variations13. The measured crater depths shown represents the characteristic depth of each crater, and the bars in Fig.2 demonstrate that this is not the case. Our pure-ice ◦ represent the azimuthal variability in the depth measurement simulations indicate that at latitudes ≤30 (Ts ∼ 150–160 K), (see Methods). viscous relaxation in a pure-ice layer would result in present- All but one of Ceres' large craters have da ≥ 2 km, and seven day apparent crater depths of less than 500 m, even if ice grains (Kerwan, Yalode, Urvara, Vinotonus, Zadeni, Chaminuka and are large (that is, maximizing the ice viscosity) and the craters Ezinu) have da ≥ 5 km. These seven craters range in diameter from formed recently (100 Myr ago). At these surface temperatures, a 116 km to 280 km. Only one crater has a characteristic apparent 100-km-diameter crater can fully relax (da ∼ 0) on timescales of ◦ > depth of less than 2 km: Coniraya (40 N, D D 135 km, da D 500 m 1–10 Myr. There are six craters with D 75 km in this latitude band Supplementary Fig. 7). This crater is discussed in detail below. on Ceres (Fig.2). All have a characteristic da ≥ 2 km, which is Many of Ceres' deep craters appear fairly young (for example, much deeper than expected for a pure-ice layer. The crater Dantu Supplementary Figs 3–5, 9 and 11); however, the crater Vinotonus (DD126 km, Supplementary Fig. 9) at 24◦ N, for example, has ◦ ◦ (DD140 km) is superposed by numerous interior craters, including da D4 km. At higher latitudes (30 –50 ) our simulations for a pure- a 46-km-diameter crater that has destroyed a portion of its southern ice layer indicate that crater topography will still viscously relax rim.
Details
-
File Typepdf
-
Upload Time-
-
Content LanguagesEnglish
-
Upload UserAnonymous/Not logged-in
-
File Pages6 Page
-
File Size-