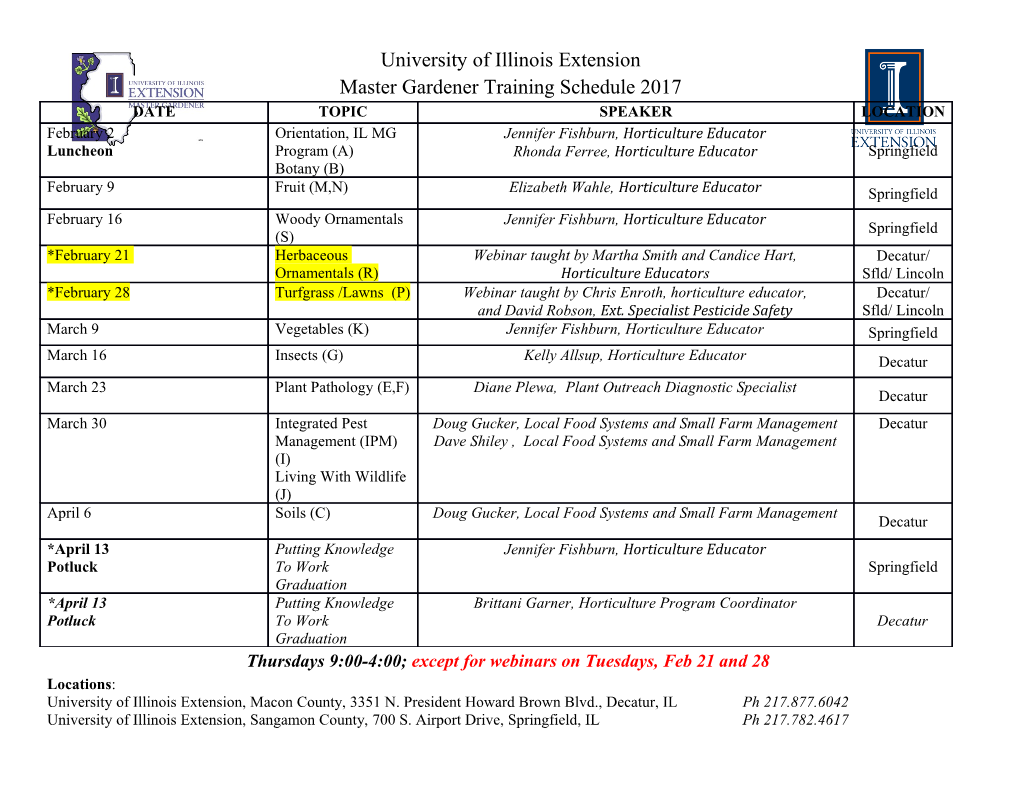
The Cryosphere, 14, 445–459, 2020 https://doi.org/10.5194/tc-14-445-2020 © Author(s) 2020. This work is distributed under the Creative Commons Attribution 4.0 License. Soil moisture and hydrology projections of the permafrost region – a model intercomparison Christian G. Andresen1,2, David M. Lawrence3, Cathy J. Wilson2, A. David McGuire4, Charles Koven5, Kevin Schaefer6, Elchin Jafarov6,2, Shushi Peng7,18, Xiaodong Chen8,19, Isabelle Gouttevin9,10, Eleanor Burke11, Sarah Chadburn12, Duoying Ji13, Guangsheng Chen14, Daniel Hayes15, and Wenxin Zhang16,17 1Department of Geography, University of Wisconsin–Madison, Madison, Wisconsin, USA 2Earth and Environmental Science Division, Los Alamos National Laboratory, Los Alamos, New Mexico, USA 3National Center for Atmospheric Research, Boulder, Colorado, USA 4Institute of Arctic Biology, University of Alaska Fairbanks, Fairbanks, Alaska, USA 5Climate and Ecosystem Sciences Division, Lawrence Berkeley National Laboratory, Berkeley, California, USA 6Institute of Arctic and Alpine Research, University of Colorado Boulder, Boulder, Colorado, USA 7Laboratoire de Glaciologie et Géophysique de l’Environnement (LGGE), Université Grenoble Alps and CNRS, Grenoble, France 8Department of Civil and Environmental Engineering, University of Washington, Seattle, Washington, USA 9IRSTEA-HHLY, Lyon, France 10IRSTEA-ETNA, Grenoble, France 11Met Office Hadley Centre, Exeter, UK 12School of Earth and Environment, University of Leeds, Leeds, UK 13College of Global Change and Earth System Science, Beijing Normal University, Beijing, China 14Environmental Sciences Division, Oak Ridge National Laboratory, Oak Ridge, Tennessee, USA 15School of Forest Resources, University of Maine, Maine, USA 16Department of Physical Geography and Ecosystem Science, Lund University, Lund, Sweden 17Center for Permafrost (CENPERM), Department of Geosciences and Natural Resource Management, University of Copenhagen, Copenhagen, Denmark 18College of Urban and Environmental Sciences, Peking University, No. 5 Yiheyuan Road, Haidian District, Beijing 100871, China 19Atmospheric Sciences and Global Change Division, Pacific Northwest National Laboratory, Richland, WA, USA Correspondence: Christian G. Andresen ([email protected]) Received: 12 June 2019 – Discussion started: 9 July 2019 Revised: 24 November 2019 – Accepted: 13 December 2019 – Published: 5 February 2020 Abstract. This study investigates and compares soil mois- plained by infiltration of moisture to deeper soil layers as ture and hydrology projections of broadly used land models the active layer deepens or permafrost thaws completely. Al- with permafrost processes and highlights the causes and im- though most models agree on drying, the projections vary pacts of permafrost zone soil moisture projections. Climate strongly in magnitude and spatial pattern. Land models tend models project warmer temperatures and increases in pre- to agree with decadal runoff trends but underestimate runoff cipitation (P ) which will intensify evapotranspiration (ET) volume when compared to gauge data across the major Arc- and runoff in land models. However, this study shows that tic river basins, potentially indicating model structural limita- most models project a long-term drying of the surface soil tions. Coordinated efforts to address the ongoing challenges (0–20 cm) for the permafrost region despite increases in the presented in this study will help reduce uncertainty in our ca- net air–surface water flux (P -ET). Drying is generally ex- pability to predict the future Arctic hydrological state and as- Published by Copernicus Publications on behalf of the European Geosciences Union. 446 C. G. Andresen et al.: Soil moisture and hydrology projections of the permafrost region sociated land–atmosphere biogeochemical processes across how differences in process representation affect projections spatial and temporal scales. of permafrost landscapes. Upgrades in permafrost representation such as freeze and thaw processes in the land component of Earth system mod- els have improved understanding of the evolution of hydrol- ogy in high northern latitudes. Particularly, soil thermal dy- 1 Introduction namics and active-layer hydrology upgrades include the ef- fects of unfrozen water on phase change, insulation by snow Hydrology plays a fundamental role in permafrost land- (Peng et al., 2016), organic soils (Jafarov and Schaefer, 2016; scapes by modulating complex interactions among biogeo- Lawrence et al., 2008) and the hydraulic properties of frozen chemical cycling (Frey and Mcclelland, 2009; Newman soils (Swenson et al., 2012). Nonetheless, large discrepan- et al., 2015; Throckmorton et al., 2015), geomorphology cies in projections remain as the current generation of mod- (Grosse et al., 2013; Kanevskiy et al., 2017; Lara et al., 2015; els substantially differ in soil thermal dynamics (e.g., Peng et Liljedahl et al., 2016), and ecosystem structure and func- al., 2016; Wang et al., 2016). In particular, variability among tion (Andresen et al., 2017; Avis et al., 2011; Oberbauer et current models’ simulations of the impact of permafrost thaw al., 2007). Permafrost has a strong influence on hydrology on soil water and hydrological states is not well documented. by controlling surface and subsurface distribution and the Therefore, in this study we analyze the output of a collection storage, drainage and routing of water. Permafrost prevents of widely used permafrost-enabled land models. These mod- vertical water flow, which often leads to saturated soil con- els participated in the Permafrost Carbon Network Model ditions in continuous permafrost while confining subsurface Intercomparison Project (PCN-MIP; McGuire et al., 2018, flow through perennially unfrozen zones (a.k.a. taliks) in dis- 2016) and contained the state-of-the-art representations of continuous permafrost (Jafarov et al., 2018; Walvoord and soil thermal dynamics in high latitudes at that time. In par- Kurylyk, 2016). However, with the observed (Streletskiy et ticular, we assess how changes in active-layer thickness and al., 2008) and predicted (Slater and Lawrence, 2013) thawing permafrost thaw influence near-surface soil moisture and hy- of permafrost, there is a large uncertainty in the future hydro- drology projections under climate change. In addition, we logical state of permafrost landscapes and in the associated provide comments on the main gaps and challenges in per- responses such as the permafrost carbon–climate feedback. mafrost hydrology simulations and highlight the potential The timing and magnitude of the permafrost carbon– implications for the permafrost carbon–climate feedback. climate feedback is, in part, governed by changes in sur- face hydrology, through the regulation by soil moisture of the form of carbon emissions from thawing labile soils and 2 Methods microbial decomposition as either CO2 or CH4 (Koven et al., 2015; Schädel et al., 2016; Schaefer et al., 2011). The impact 2.1 Models and simulation protocol of soil moisture changes on the permafrost carbon feedback could be significant. Lawrence et al. (2015) found that the This study assesses a collection of terrestrial simulations impact of the soil drying projected in simulations with the from models that participated in the PCN-MIP (McGuire Community Land Model decreased the overall global warm- et al., 2018, 2016; Table 1). The analysis presented here is ing potential of the permafrost carbon–climate feedback by unique as it focuses on the hydrological component of these 50 %. This decrease was attributed to a much slower increase models. Table 2 describes the main hydrological characteris- in CH4 emissions if surface soils dry, which is partially com- tics for each model. Additional details on participating mod- pensated for by a stronger increase in CO2 emissions under els regarding soil thermal properties, snow, soil carbon and drier soil conditions. forcing trends can be found in previous PCN-MIP studies Earth system models project an intensification of the hy- (e.g., McGuire et al., 2016; Koven et al., 2015; Wang et al., drological cycle characterized by a general increase in the 2016; Peng et al., 2016). It is important to note that the ver- magnitude of water fluxes (e.g., precipitation, evapotranspi- sions of the models presented in this study are from McGuire ration and runoff) in northern latitudes (Rawlins et al., 2010; et al. (2016, 2018) and some additional improvements to in- Swenson et al., 2012). In addition, intensification of the hy- dividual models may have been made since then. drological cycle is likely to modify the spatial and tempo- The simulation protocol is described in detail in McGuire ral patterns of water in the landscape. However, the spatial et al. (2016, 2018). In brief, models’ simulations were con- variability, timing and reasons for future changes in hydrol- ducted from 1960 to 2299, partitioned by historic (1960– ogy in terrestrial landscapes in the Arctic are unclear, and 2009) and future simulations (2010–2299), where future variability in projections of these features by current terres- simulations were forced with a common projected climate trial hydrology applied in the Arctic has not been well doc- derived from a fully coupled climate model simulation umented. Therefore, there is an urgent need to assess and (CCSM4; Gent et al., 2011). Historic atmospheric forcing better understand hydrology simulations in land models and datasets (Table 1; e.g., climate, atmospheric CO2, N depo- The Cryosphere, 14, 445–459, 2020 www.the-cryosphere.net/14/445/2020/
Details
-
File Typepdf
-
Upload Time-
-
Content LanguagesEnglish
-
Upload UserAnonymous/Not logged-in
-
File Pages15 Page
-
File Size-