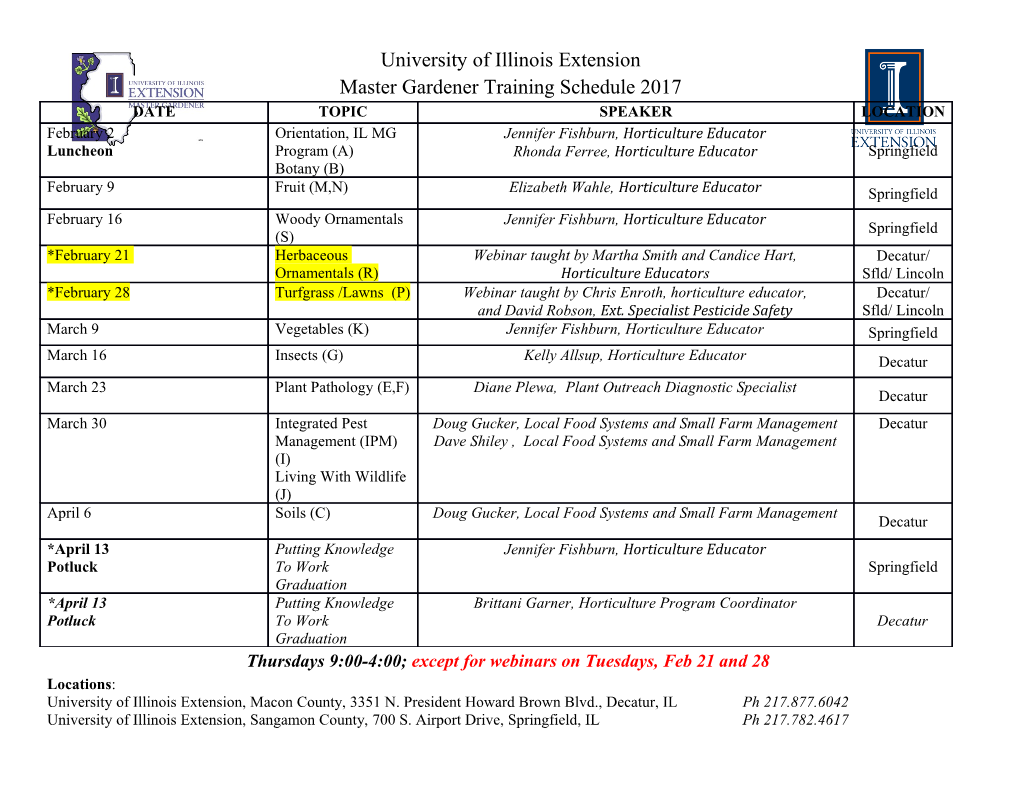
BUNDLES AND BARRELS 5 A. B. Structures of helical bundle and β-barrel membrane proteins differ in many respects, seen in the ribbon diagrams of the photosynthetic reaction center from Rb. sphaeroides (a) and the maltoporin trimer from E. coli outer membrane (b). (a) Redrawn from Jones, M. R., et al., Biochim Biophys Acta. 2002, 1565: 206–214. © 2002 by Elsevier. Reprinted with permission from Elsevier; (b) redrawn from Wimley, W. C., Curr Opin Struct Biol. 2003, 13:404–411. © 2003 by Elsevier. Reprinted with permission from Elsevier. The thermodynamic arguments discussed in the pre- HELICAL BUNDLES vious chapter make it clear that the TM segments of proteins will utilize secondary structure to satisfy the Transmembrane (TM) α-helices have dominated the pic- hydrogen bond needs of the peptide backbone. While a ture of membrane proteins, guided by early structural variety of combinations of secondary structures might information on bacteriorhodopsin and by the first x-ray be imagined in polytopic membrane proteins, all known structure solved for membrane proteins, that of the pho- protein structures cross the bilayer with either α-heli- tosynthetic reaction center (RC). The majority of integral ces or β-strands. This produces two classes of integral membrane proteins whose high-resolution structures membrane proteins: helical bundles and β-barrels. This have been solved by x-ray crystallography exhibit the chapter looks at the structure and function of the para- helical bundle motif (see many examples in Chapters 9 digmatic proteins in each class, then covers the surpris- through 13). Helix–helix interactions have been analyzed ing variation among β-barrels, and ends with a look at in many of these, providing details of both tertiary and proteins that fit neither class. quaternary interactions. Identification of new integral 107 Bundles and barrels membrane proteins in the proteome relies heavily on Flagella prediction of TM helices, as described in Chapter 6. ϩ ϩ ϩϩϩ ϩ ϩ ϩ ϩ Bacteriorhodopsin ϩ ϩ Ϫ Ϫ ϩ If a single protein dominated the thinking about struc- ϩ Ϫ Ϫ ϩ ture, dynamics, and assembly of membrane proteins in Hϩ ϩ Ϫ ADP ϩ P Ϫ ϩ the decades following 1970, that protein was bacteriorho- ϩ Ϫ i Ϫ ϩ ATP dopsin (bR) from the purple membranes of the salt-loving ϩ Ϫ ϩ bacterium Halobacter salinarum. From early structural Light ϩ Ϫ ϩ Hϩ images and spectroscopic characterizations, bR became ϩ Ϫ ATPase ϩ the paradigm for ion transport proteins and indeed for ϩ ϪϪϩ α-helical TM proteins in general. From the wealth of stud- ϩ Ϫ Ϫ ϩ ies of its structure and function, a truly detailed under- Purple ϩ Ϫ Ϫ ϩ standing of this membrane protein has emerged. membrane ϩ Ϫ ϩ Hϩ ATPase bR is the only protein species in the discrete mem- ϩ Ϫ ϩ brane domains called purple membranes, the light-sen- ϩ Ϫ ϩ ATP sitive regions of the plasma membranes of H. salinarum ϩ Ϫ ϩ Ϫ ϩ ADP Pi (Figure 5.1). Together with specialized lipids, this pro- ϩ Ϫ Ϫ ϩ tein forms functional trimers that pack as ordered Red ϩ Ϫ Ϫ ϩ Respiratory membrane two-dimensional arrays on the bacterial cell. In pho- ϩ Ϫ ϩ chain tophosphorylation bR functions to pump protons out ϩ Ϫ ϩ O2 of the cell in response to the absorption of light by its ϩ Ϫ ϩ chromophore, retinal, converting light energy into an ϩ ϪϪSubstrate ϩ Cell Hϩ electrochemical proton gradient that supports the syn- ϩ Ϫ Ϫ ϩ wall Ϫ Ϫ thesis of ATP. The ability of reconstituted vesicles con- ϩ Ϫ Ϫ Ϫ Ϫ ϩ taining bR and beef heart mitochondrial ATP synthase ϩ ϩ to synthesize ATP in response to light provided crucial ϩ ϩϩϩ ϩ ϩ early support for Mitchell’s chemiosmotic hypothesis Flagella that the energy of an electrochemical gradient across the membrane could be used to do work (Figure 5.2). 5.1 Schematic showing the different membrane domains Like rhodopsin, the light-absorbing protein in the rod of a halobacterium cell. The patches of purple membrane outer segments of the eye’s retina (see Chapter 10), bR containing bacteriorhodopsin (bR) are separate from the has seven helices labeled A to G that span the membrane, regions of membrane containing the respiratory chain and and a retinal that is bound to a lysine residue in helix G the ATP synthase. Protons are pumped out of the cell in via a protonated Schiff base (Figure 5.3). Whereas bR response either to light absorption by bR in the purple undergoes a light-induced photocycle involving conver- membrane or to cytosolic substrates for the electron sion of the retinal from all-trans to 13-cis accompanied transport chain. The ATP synthase normally uses the by conformational changes in the protein that result in uptake of protons to drive the synthesis of ATP, although proton transfer across the membrane, visual rhodopsin’s it can act as an ATPase and eject protons at the expense activation cascade involves an 11-cis to all-trans conver- of ATP. Redrawn from Stoeckenius, W., Sci Am. 1976, sion of the retinal, followed by its dissociation from the 234:38–46. © 1976 by Scientific American. Reprinted with protein. In addition to bR, H. salinarum contains three permission from Scientific American. related rhodopsins, and similar molecules are found in eubacteria and unicellular eukaryotes. The purple membrane is composed of 75% protein bR was the first integral membrane protein whose and 25% lipid by weight, with ten halobacterial lip- topological organization in the membrane was eluci- ids per bR monomer. These native lipids are based on dated. Electron diffraction of the native two-dimensional archeol (see Figure 2.6), so they differ in headgroups but crystalline arrays of purple membrane provided early not in length of acyl chains. Delipidation by treatment images of bR trimers, revealing the monomer structure with a mild detergent affects the kinetics of the bR reac- to be seven TM α-helices arranged in an arc-like double tion; addition of halobacterial lipids but not phospholip- crescent in the plane of the bilayer (Figure 5.4a,b). Mod- ids restores activity. When bR is crystallized (see below) els fitting the primary structure of the protein, with 70% the bound lipids that are retained from the membrane of its 248 residues being hydrophobic, to the observed fit well into the grooves along the protein surface (see images were aided by the sensitivity of exposed loop resi- Figure 8.20). dues to partial proteolysis in situ (carried out on bR in 108 Helical bundles C18 C20 Light C19 ϩ C5 C7 C9 C11 C13 C15 C⑀ Lys216 C4 C6 C8 C10 C12 C14 N Hϩ C3 C1 H C2 C17 C16 h Bacteriorhodopsin C18 C19 C20 C5 C7 C9 C11 C13 C4 C6 C8 C10 C12 C14 ϩ C3 C1 C15 H C17 C2 N C16 Hϩ C⑀ Lys216 5.3 Retinal bound to lysine 216 in bacteriorhodopsin. The Schiff base linkage (shaded) between the aldehyde of retinal and the ε-amino group of lysine 216 is protonated, as shown, before light stimulation. The all-trans retinal converts to 13-cis retinal upon absorption of a photon. Redrawn from Neutze, R., et al., Biochim Biophys Acta. 2002, 1565:144–167. © 2002 by Elsevier. Reprinted with permission from Elsevier. Mitochondrial F1F0-ATP synthase Lipid Asp85 and Asp96. These results led to a model for the vesicle topology of bR that was further modified as genetic stud- ies defined the positions of many residues (Figure 5.4c). Extensive mutagenesis of the gene for bR provided a large collection of mutant proteins that could be studied in cell suspensions or reconstituted in lipid vesicles, with changes of pH, temperature, and salt conditions used to further characterize the protein function. In addition, a ADP ϩ P ATP variety of retinal analogs were incorporated to observe Hϩ their effects on the absorption spectrum and activ- ity. Researchers used a number of pH-sensitive dyes to 5.2 Schematic of reconstituted vesicles containing investigate the stoichiometry of proton pumping. Over bR and ATP synthase. When the light is turned on, ATP many years, increasingly sophisticated instrumentation is synthesized from ADP + Pi. When the light is turned for visible and ultraviolet absorbance, fluorescence, cir- off, ATP synthesis stops. These vesicles gave important cular dichroism, Raman, and infrared spectroscopy have evidence to support the chemiosmotic theory of Peter been employed to follow the response of bR to light. Mitchell. Redrawn from Garrett, R. H., and C. M. Grisham, The primary event when bR absorbs a photon is the Biochemistry, 2nd ed., Brooks/Cole, 1999, p. 897. isomerization of retinal. This event triggers subsequent © 1999. Reprinted with permission from Brooks/Cole, a structural changes and pK shifts in the protein that division of Thomson Learning: www.thomsonrights.com. a allow deprotonation of the Schiff base, vectorial transfer of the proton to the extracellular side of the membrane, the membrane), although the precise beginning and end and uptake of a proton from the cytosol. These processes of each helix were uncertain for years. Such studies also are accompanied by differences in the absorbance spec- showed that a few N-terminal amino acids are exposed trum of bR, allowing detection of intermediates with to the exterior and the last 17 to 24 amino acids of the lifetimes varying from a few picoseconds (ps, 10−12 sec) C terminus are accessible in the cytoplasm. The loca- to a few milliseconds (msec, 10−3 sec). tion of retinal in the center of the protein (Figure 5.4b) The light-induced changes in bR are summarized in was determined by neutron diffraction, and the lysine a photoreaction cycle, or photocycle (Figure 5.5).
Details
-
File Typepdf
-
Upload Time-
-
Content LanguagesEnglish
-
Upload UserAnonymous/Not logged-in
-
File Pages101 Page
-
File Size-