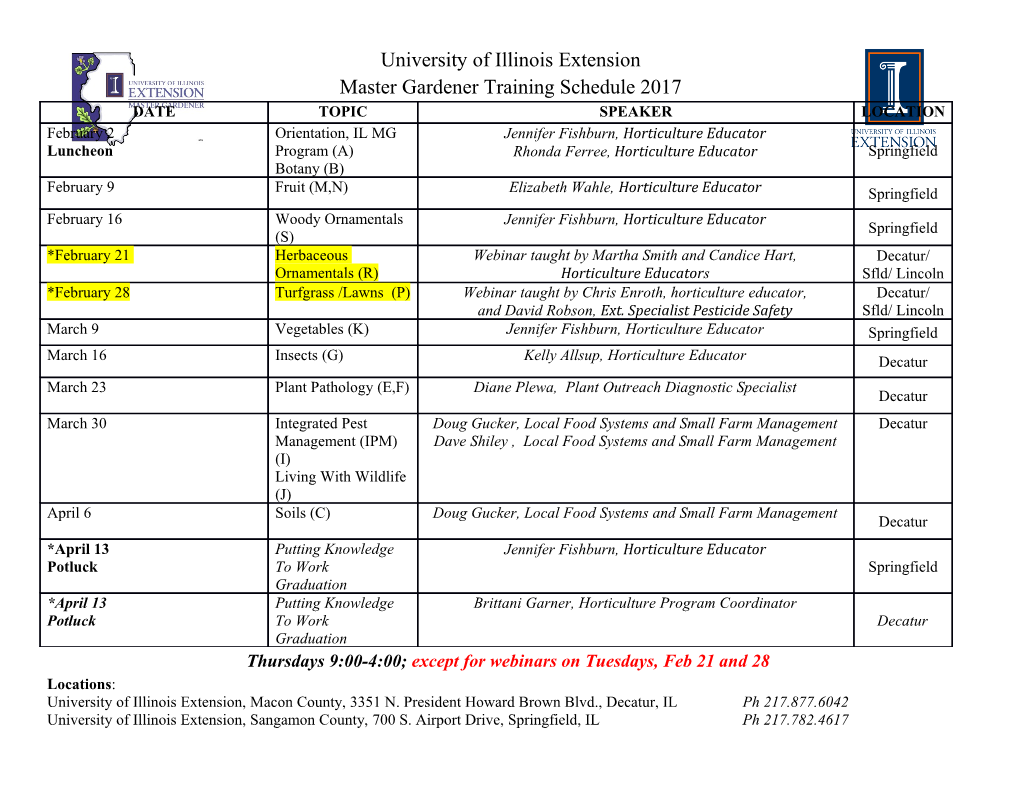
manuscript submitted to Geophysical Research Letters 1 Forcing of the Preferred Positions of the North 2 Atlantic Eddy-driven Jet: from Above and Below 1,2 2 3 3 Rachel H. White , Casey Hilgenbrink , Aditi Sheshadri 1 4 Barcelona Supercomputing Center, 08034 Barcelona, Spain 2 5 University of Washington, Seattle, WA 98195 3 6 Stanford University, Stanford, CA 94305, USA 7 Key Points: 8 • Greenland orography creates the northern preferred latitude of the North Atlantic 9 jet latitude index by forcing Greenland tip jet events 10 • Forced meridional shifts in the North Atlantic jet typically manifest as changes 11 in the probability of preferred latitudes 12 • CMIP5 biases in simulating a northern preferred latitude are connected to clima- 13 tological jet position biases Corresponding author: Rachel H. White, [email protected] –1– manuscript submitted to Geophysical Research Letters 14 Abstract 15 The atmospheric eddy-driven jet over the North Atlantic exhibits three ‘preferred po- 16 sitions’, latitudes where the jet maximum occurs more frequently than others. Using a 17 state-of-the-art dynamical atmosphere model (WACCM), we explore the forcing of these 18 preferred positions by upper-atmosphere circulation and northern hemisphere mountain 19 ranges. The latitude of the northern preferred position shifts only when the latitudinal 20 position of Greenland is changed, and this preferred position disappears when Green- 21 land orography is flattened. We propose that Greenland tip jet events create the appear- 22 ance of an eddy-driven jet northern ‘preferred position’. In ERA-interim data, years with 23 a higher frequency of tip jet events show a higher frequency of the northern preferred 24 position. Additionally, we show that CMIP5 models underestimate the frequency of the 25 northern preferred position when the climatological jet is too narrow or biased too far 26 south. This is a cautionary tale of the interpretation of results from reducing the dimen- 27 sionality of data. 28 1 Introduction 29 Amongst the intrinsic variability of our weather, there is evidence of patterns that 30 show anomalously high persistence or occurrence relative to other states (Rex, 1950; Namias, 31 1964; Reinhold & Pierrehumbert, 1982; Branstator, 1987; Vautard & Legras, 1988; Vau- 32 tard, 1990; Hannachi et al., 2017). These are known as weather regimes, and can be con- 33 sidered attractor states (Marshall & Molteni, 1993; Crommelin et al., 2004). Extreme 34 weather events have been related to weather regimes (Franzke, 2013; Coumou et al., 2014; 35 Hoskins & Woollings, 2015; Grotjahn et al., 2016; Messori et al., 2018); understanding 36 the underlying dynamics of such regimes may therefore improve our understanding of 37 the occurrence of extreme events. There is, however, a lack of consensus on even the op- 38 timum number of regimes required to characterize observed atmospheric non-Gaussian 39 behaviour (Hannachi et al., 2017), suggesting that much progress remains to be made 40 on understanding regime behaviour in our atmosphere. 41 Atmospheric blocking is a weather regime during which regions of high pressure 42 remain quasi-stationary (Rex, 1950; Woollings et al., 2018), associated with weather anoma- 43 lies both locally and downstream (Carrera et al., 2004; Bao & Wallace, 2015), includ- 44 ing extreme events (Carrera et al., 2004; Horton et al., 2016; Lau & Kim, 2011). A block- 45 ing event in Spring 2018, dubbed the Beast from the East in the media, was associated 46 with extreme cold temperatures over Northern Europe. Blocks in di↵erent locations have 47 been associated with di↵erent weather regimes. In the northern hemisphere atmospheric 48 blocks tend to occur over the north-east Atlantic/western Europe or over the North Pa- 49 cific (Barriopedro et al., 2006; Dunn-Sigouin & Son, 2013). North-east Atlantic/Europe 50 blocking is often subcategorized into Greenland blocking, and European (or Scandina- 51 vian) blocking (Barriopedro et al., 2006; Madonna et al., 2017). 52 Over the North Atlantic region, the North Atlantic Oscillation (NAO) is the strongest 53 mode of variability (Wallace & Gutzler, 1981), associated with meridional shifts of the 54 low-level, eddy-driven jet (Eichelberger & Hartmann, 2007). The probability density func- 55 tion of the NAO index is negatively skewed, suggesting the existence of two regimes: one 56 blocking regime (negative NAO), and a zonal (or no-blocking) flow regime (Woollings, 57 Charlton-Perez, et al., 2010). Rossby wave breaking has been proposed as a potential 58 mechanism for this NAO regime behaviour (Hannachi, 2007; Woollings et al., 2008). 59 Recently, Woollings, Hannachi, and Hoskins (2010) identified three preferred po- 60 sitions of the Atlantic eddy-driven jet by defining a relatively simple jet latitude index 61 (JLI); this index finds the latitude of the maximum value in daily Atlantic low-level zonal 62 mean zonal wind. The probability density function of this index shows three distinct lat- 63 itude peaks, or preferred positions of the jet. These preferred positions are unique to the 64 North Atlantic sector and are most distinct in winter (Woollings, Hannachi, & Hoskins, –2– manuscript submitted to Geophysical Research Letters 65 2010; Woollings et al., 2018). Many general circulation models (GCMs) are unable to 66 reproduce this observed behaviour of the jet (Anstey et al., 2013; Hannachi et al., 2013; 67 Iqbal et al., 2017), limiting our ability to understand future changes in this behavior in 68 a warming climate. 69 The southern preferred position of the JLI has been connected with a previously 70 identified weather regime, Greenland blocking (Madonna et al., 2017; Hannachi et al., 71 2012); however, a conclusive explanation for the separation of the central and northern 72 preferred positions has remained elusive. Correlations exist between the northern peak 73 and both the NAO, and the Eastern Atlantic pattern (Franzke, 2013; Woollings et al., 74 2018; Hannachi et al., 2012), as well as with European blocking (Davini, Cagnazzo, Fogli, 75 et al., 2014), and anti-cyclonic vs. cyclonic Rossby wave-breaking has been suggested as 76 a mechanism for producing these preferred positions (Franzke, 2013; Woollings et al., 2011). 77 In this study we use re-analysis data and atmospheric GCM simulations to study 78 the response of the preferred positions of the Atlantic eddy-driven jet to forcing from the 79 stratosphere and to the presence/absence of orography. We propose a mechanism for the 80 separation of the distinct northern preferred position from the central position, based 81 on Greenland orography. In the framework of this mechanism we present evidence that 82 biases in the simulation of the eddy-driven jet mean climatological position and width 83 can explain GCMs biases in the frequency of the northern preferred position. 84 2 Model and Methods 85 2.1 Data, model, and simulations 86 Analysis of the JLI requires daily troposphere zonal wind data across the Atlantic 87 sector at all latitudes for many years, which are unavailable in true observations; we thus 88 use ERA-Interim (ERA-I) re-analysis data (Dee et al., 2011). To extend beyond that which 89 can be gleaned from observations, we perform simulations using the Whole Atmosphere 90 Community Climate Model 4 with Specified Chemistry (WACCM-SC) (Marsh et al., 2013), 91 with a horizontal resolution of 2.5◦longitude by 1.9◦latitude. The observed climatology 92 and variability of atmospheric circulation, including the stratosphere, is reproduced well 93 by the WACCM (Marsh et al., 2013; de la Torre et al., 2012). In our simulations, sea 94 surface temperatures (SSTs) and sea ice fraction are fixed to observed values from the 95 merged Hadley NOAA Optimum Interpolation (NOAA/OI) data set (Hurrell et al., 2008). 96 For comparison to ERA-I, simulations are run with observed monthly SSTs from 1979- 97 2010 (WACCM CTL); while monthly climatological SSTs (1982-2001) are used for all 98 other experiments to allow for longer simulations of 60 years while avoiding any climate 99 change trends. The first year is discarded as spin-up. In all simulations atmospheric con- 100 stituents (greenhouse gases, aerosols) are fixed to year 2000 levels. 101 To explore the response of the Atlantic JLI to orography (large-scale mountain ranges 102 and ice sheets), four main experiments are performed: Flat - all global orography is flat- 103 tened, No H, - the Himalaya are flattened, including the Tibetan plateau and more north- 104 ern Mongolian mountains; No R - the North American Rockies are flattened; and No G 105 - Greenland orography is flattened. Supplemental figure S1 shows the lower boundary 106 condition surface height for each experiment. Where orography is flattened the surface 107 height is reduced to 50 m above sea level, and variables describing sub-grid scale oro- 108 graphic variability are reduced to typical values of low-lying land; see White et al. (2017) 109 for further details. 110 2.2 Jet Latitude Index and uncertainty estimates 111 To calculate the JLI, daily 850mb zonal winds are zonally averaged over the North 112 Atlantic sector (60◦W-0◦W; 15◦N - 75◦N) following Woollings, Hannachi, and Hoskins –3– manuscript submitted to Geophysical Research Letters 113 (2010). Cubic splines are used to interpolate the meridional profile of daily zonal-mean 114 zonal wind in a 3 latitude band centered on the maximum value. The jet latitude for a 115 given day is the latitude at which the maximum value of this interpolant occurs. The 116 probability density function (PDF) of this JLI is estimated using a kernel density esti- 117 mation method (Silverman, 1981). 118 We estimate uncertainty on our JLI PDFs through a bootstrap resampling approach 119 (Efron & Tibshirani, 1986): we construct a synthetic jet latitude timeseries by randomly 120 sampling (with replacement) n seasons from the jet latitude timeseries, where n is the 121 number of seasons in the original timeseries.
Details
-
File Typepdf
-
Upload Time-
-
Content LanguagesEnglish
-
Upload UserAnonymous/Not logged-in
-
File Pages18 Page
-
File Size-