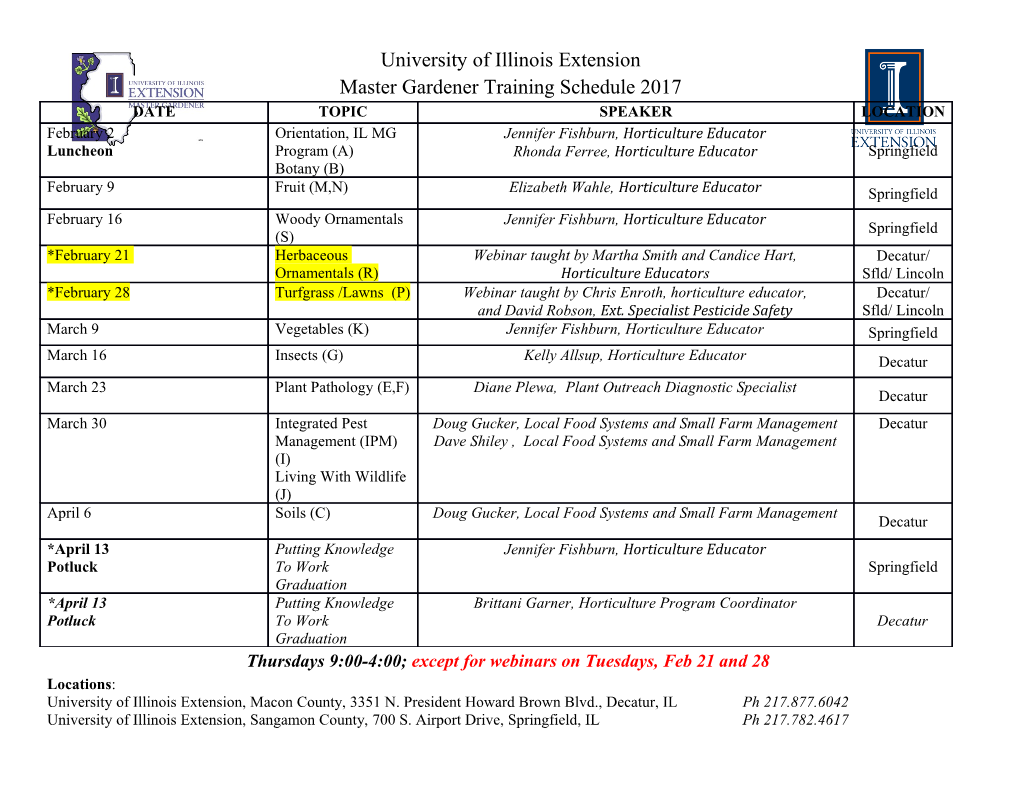
Isotope Geochemistry W, M. White Chapter 11 UNCONVENTIONAL ISOTOPES AND APPROACHES 11.1 INTRODUCTION Traditional stable isotope geochemistry, the field developed by Harold Urey and his students, fo- cused on simple isotope ratios of light elements that can be analyzed in a gas source mass spectrometer: 1H/2H, 13C/12C, 15N/14N, 18O/16O, and 34S/32S. Over the past decade or two, stable isotope geochemistry has expanded in three important new directions. First, geochemists have begun to exploit the tendency of isotopes of pairs of elements, particularly carbon and oxygen, to “clump” in thermodynamically preferred isotopologues. We introduced the theoretical basis of this approach in Chapter 8. In this chapter, we explore some examples of the uses and applications of this approach. Second, by analyzing additional isotope ratios of oxygen and sulfur, specifically 17O/16O and 33S/32S, mass-independent fractionations were discovered in terrestrial materials in the past decade or so. As we found in Chapter 8, both equilibrium and kinetic effects should produce fractionations whose mag- nitude depends on the relative mass difference between the isotopes of interest; this is known as mass- dependent fractionation. However, reactions induced by relatively high-energy photons in the atmos- phere can produce fractionations in which the magnitude of fractionation is independent of the mass difference between isotopes. Studying these mass-independent fractionations have led to important new insights into Earth processes and evolution. Third, the list of elements of interest has greatly expanded, including both light elements such as Li and B, which we can expect will experience relatively large fractionations, but also heavier metals and gases such as Mg, Si, Cl, Ca, Fe, Cu, Zn, Se, Mo, Tl Hg, and U, which experience smaller, but nonethe- less significant fractionations. A variety of techniques are used in analysis including thermal ionization + (for example, B can be analyzed as the heavy ion CsBO2 ), gas source mass spectrometry (Cl), multi- Table 11.1. Values of Non-Conventional Stable Isotope Ratios Element Notation Ratio Standard Absolute Ratio Lithium δ7Li 7Li/6Li NIST L-SVEC 12.1735 Boron δ11B 11B/10B NIST 951 4.0436 Magnesium δ26Mg 26Mg/24Mg DSM3 0.13979 Silicon δ30Si 30Si/28Si NBS28 (NIST-RM8546) 0.033532 δ29Si 29Si/28Si 0.050804 Chlorine δ37Cl 37Cl/35Cl seawater (SMOC) 0.31963 NIST-SRM 975 0.31977 Calcium δ44/42Ca 44Ca/42Ca NIST SRM 915a 0.310163 δ44/40Ca 44Ca/40Ca 0.021518 δ43/42Ca 43Ca/42Ca 0.208655 Iron δ56Fe 56Fe/54Fe IRMM-14 15.698 δ57Fe 57Fe/54Fe 0.363255 Copper δ65Cu 65Cu/63Cu NIST 976 0.44562 Zinc δ68Zn 68Zn/64Zn JMC3-0749L 0.37441 δ66Zn 68Zn/64Zn 0.56502 Molybdenum δ97/95Mo 97Mo/95Mo various* 0.5999 δ98/95Mo 98Mo/95Mo 1.5157 *No interlaboratory standard has been agreed upon. Based on data listed in Coplen et al. (2002). 327 1/9/13 Isotope Geochemistry W, M. White Chapter 11 collector ICP-MS, and ion probe techniques. The theoretical principles are the same as we discussed in Chapter 8: heavier isotopes will enter the phase with the stronger bond and the extent of fractionation depends on temperature. The largest fractionations will occur where the element is partitioned be- tween phases in which the element’s atomic environment is very different or its oxidation state changes. Biologically mediated reactions produce some of the largest fractionations for these new ele- ments, particularly Fe, Cu, and Zn, just as was the case for traditional elements C, N, and S. The list of these “non-traditional” stable isotopes is sufficiently long that we have space here only to “highlight” some of the more fascinating insights these studies have provided. More details on these elements can be found the Volume 55 of Reviews in Mineralogy: Geochemistry of Non-Traditional Stable Isotopes (John- son et al., 2004). These non-traditional stable isotopes are summarized in Table 11.1. 11.2 APPLICATIONS OF ISOTOPIC CLUMPING We introduced the theory behind isotopic “clumping” studies in Chapter 8. Here we’ll consider ap- plications to geothermometry and paleoclimatology. A significant advantage of clumped isotope geo- thermometry is that, assuming equilibrium was achieved between a carbonate mineral and the water from which it precipitated and no subsequent disturbance of the system, both paleotemperatures and the isotopic composition of the water can be determined from analysis of the carbonate. This is because 13 18 16 the ∆47 parameter (a measure of the abundance of the C O O isotopologue relative to a purely sto- chastic distribution we introduced in Chapter 8) is a function only of the equilibrium temperature for a given mineral. Once the equilibrium temperature is known, then the δ18O and δ13C of the water can be calculated from the δ18O and δ13C of the carbonate (e.g., equation 10.1). We saw in Chapter 10 how oxygen and hydrogen isotope geothermometry has successful determined the cause of the Pleistocene “Ice Ages” and reconstructed climate variation through the Cenozoic. Qualitative proxies such as fossils and glacial sediments indicate that dramatic climate variations also occurred in the Mesozoic and, particularly, the Paleozoic: there is evidence of glaciation in the Carbon- iferous that was as severe as the Pleistocene as well as evidence for perhaps less severe glaciation in the Late Ordovician, with intervening periods that appear to have been significantly warmer than the pre- sent. Such warmer temperatures would be consistent with much higher modeled atmospheric CO2 con- centrations in the Paleozoic (such as shown in Figure 10.43). However, oxygen isotope geothermometry based on carbonate fossils suggests that variations of tropical sea surface temperatures were relatively was small over this time. Conventional isotope geothermometry, however, necessarily requires as- sumptions about the oxygen isotopic composition of seawater of the time. For the Pleistocene and even the Tertiary, the isotopic composition of seawater is readily constrained (as discussed in Chapter 10). This is not so easily done for periods earlier in Earth’s history. Veizer et al. (1999) argued, based on analysis of marine carbonate fossils, that δ18O increased from a mean value of about -8‰ in the Cam- brian to a present value close to 0‰. Muehlenbachs, however, has argued that seawater δ18O has been buffered by ridge-crest hydrothermal activity to values close to 0 throughout Earth’s history (Muehlen- bachs, 1976; Muehlenbachs and Furnas, 2003; Chapter 9). To address these issues, Came et al. (2007) analyzed carbon and oxygen isotopes of Silurian brachio- pods from Anticosti Island, Canada and Carboniferous (Pennsylvanian) molluscs from Oklahoma; both of these localities were tropical at the time. Came et al applied clumped isotope geothermometry to de- termine the temperatures of seawater in which they grew. Although all samples appeared to be well preserved based on various criteria, some proved to have been diagenically altered, yielding unrea- sonably high temperatures and anomalous δ13C and δ18O seawater values. The remaining samples yielded growth temperatures of 24.9±1.7 ˚C for the Carboniferous, and 34.9±0.4 ˚C for the Silurian. The Carboniferous temperatures are similar to or slightly lower than those of modern tropical seas, while the Silurian temperatures are significantly warmer. The authors point out that these temperature differ- ences are consistent with models of atmospheric CO2 during the Paleozoic: CO2 was substantially higher in the Silurian than in the both the Carboniferous and the present. Came et al. also calculated 328 1/9/13 Isotope Geochemistry W, M. White Chapter 11 18 δ OSMOW of seawater for both times and obtained values of -1.2±0.1‰ and -1.6±0.5‰, values quite close 18 to the modern one (the present seawater δ OSMOW is, of course, 0, but is ~ -1.5‰ if Antarctic and Green- land ice is included). The results suggest that the δ18O of seawater has been approximately constant, at least through the Phanerozoic, and that the low values of δ18O observed for Paleozoic carbonates by Veizer et al. (1999) appear to reflect a combination of higher seawater temperatures and, predomi- nately, diagenetic alteration of the carbonates. For the second example, we fast-forward several hundred million years to the Pliocene of East Africa, the time and place of the emergence of the genus Homo. Abundant hominid fossils and artifacts of Plio- Pleistocene age have been found in the Turkana Basin, part of the East African Rift in Kenya. This area presently is among the hottest 1% of land on the planet with a mean annual temperature of almost 30˚C. Much of the basin is occupied by a saline lake, Lake Turkana, and the surround land is quite arid. But what was the climate there like as our genus evolved from Australopithecine ancestors? Some pa- leoclimatic proxies derived from fossil pollen assemblages suggest that late Pliocene temperatures were cooler than present, while other proxies and models suggest an even warmer climate. To resolve this, Passey et al. (2010) applied clumped isotope geothermometry to paleosols of Pliocene and Pleistocene Figure 11.1. (a) ∆47 and calculated soil temperatures of paleosols from the Turkana Basin, Kenya. 13 Dashing line shows the average modern soil temperature at a depth of 50 cm. (b) Measured δ CPDB 18 and calculated δ OSMOW of Turkana Basin paleosols. Data from Passey et al. (2010). 329 1/9/13 Isotope Geochemistry W, M. White Chapter 11 age from the Turkana Basin. Before doing so, however, they demonstrated that temperatures calculated from ∆47 in modern soil carbonates did in fact reflect the climate in which they formed. They found good agreement between calculated temperatures from soil carbonates and mean annual temperatures in tropical regions, although in temperature latitudes (such as California), soil carbonate clumped iso- tope temperatures correlated with mean warm season temperature rather than mean annual tempera- ture.
Details
-
File Typepdf
-
Upload Time-
-
Content LanguagesEnglish
-
Upload UserAnonymous/Not logged-in
-
File Pages58 Page
-
File Size-