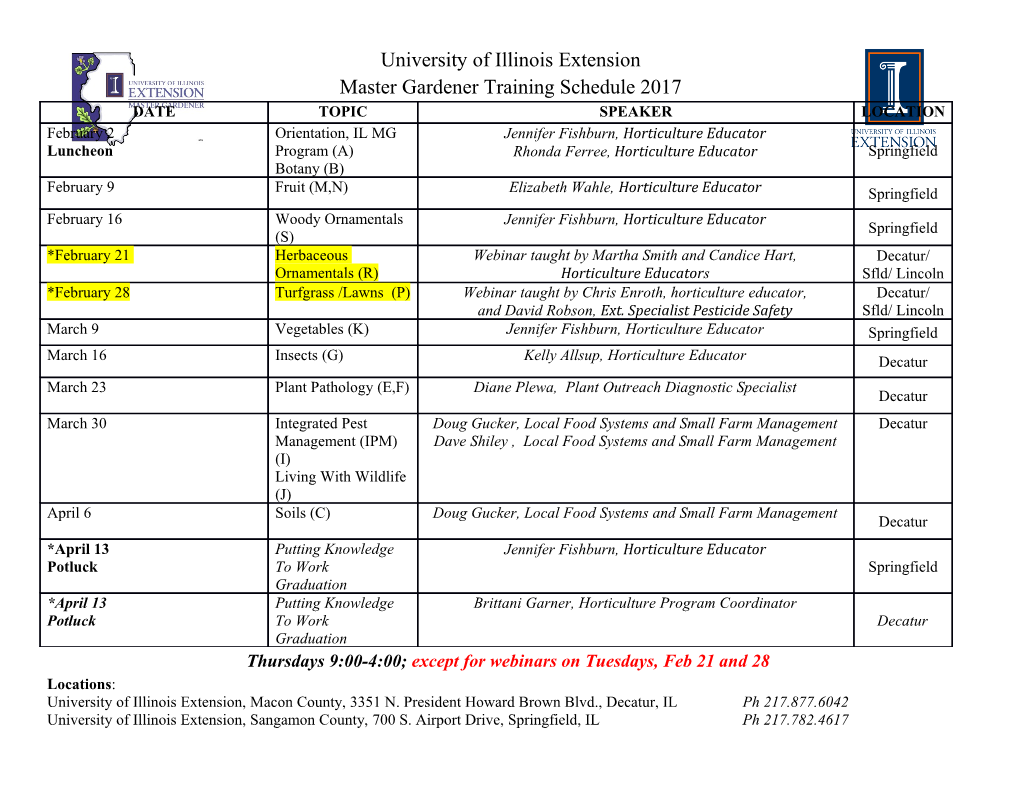
Protein Adaptation to High Hydrostatic Pressure: Computational Analysis of the Structural Proteome Samvel Avagyan1,2, Daniel Vasilchuk1,3§ and George I. Makhatadze1,2,3,* 1Center for Biotechnology and Interdisciplinary Studies, 2Department of Biological Sciences, 3Department of Chemistry and Chemical Biology, Rensselaer Polytechnic Institute, Troy, New York 12180 *Corresponding Author: George I. Makhatadze, Center for Biotechnology and Interdisciplinary Studies,, Rensselaer Polytechnic Institute, 110 8th Street, Troy, NY 12180 USA Phone: (518) 276- 4417 E-mail: [email protected] Keywords: Piezophilic adaptation; Protein stability; Protein Volume; Structural Proteome; Pressure Perturbation Calorimetry; Structural Modeling; Osmolytes - 1 - ABSTRACT Hydrostatic pressure has a vital role in the biological adaptation of the piezophiles, organisms that live under high hydrostatic pressure. However, the mechanisms by which piezophiles are able to adapt their proteins to high hydrostatic pressure is not well understood. One proposed hypothesis is that the volume changes of unfolding (ΔVTot) for proteins from piezophiles is distinct from those of non-piezophilic organisms. Since ΔVTot defines pressure dependence of stability, we performed a comprehensive computational analysis of this property for proteins from piezophilic and non-piezophilic organisms. In addition, we experimentally measured the ΔVTot of acylphosphatases and thioredoxins belonging to piezophilic and non-piezophilic organisms. Based on this analysis we concluded that there is no difference in ΔVTot for proteins from piezophilic and non-piezophilic organisms. Finally, we put forward the hypothesis that increased concentrations of osmolytes can provide a systemic increase in pressure stability of proteins from piezophilic organisms and provide experimental thermodynamic evidence in support of this hypothesis. - 2 - 1. INTRODUCTION Single-celled organisms represent the dominant form of life on earth 1. Exploration has led us to appreciate not only the diversity of life on earth, but also the diversity of the environments in which these organisms live 2. Until recently, it was thought that life could not exist in extreme conditions such as the bottom of the ocean or deep subsurface environments 2-5. However, collection of pristine samples from such environments, and exploration of deep caves and mines has shown that many life forms, including bacteria, archaea, and eukarya exist in places previously thought to be uninhabitable 2,6. Examining of the genomic data from these samples has led to estimate that the vast majority of life exists in the depths of the oceans and buried deep in the subsurface crust 1,6. Such studies have suggested that there is a trove of species living in the deepest parts of the earth’s crust waiting to be discovered. For example, it is known that the crust of the earth is not uniform and large cracks, or fissures, penetrate kilometers into the earth 2. These fissures are full of water, and because they are so deep, the heat from the mantle of the earth is able to warm these waters 2. Minerals are then able to dissolve into these waters providing the primary ingredients to sustain life 6. Interestingly, similar conditions have been proposed to be drivers of life Jupiter’s moon Europa. On Europa, it has been suggested that there is sufficient geological and tidal activity in its ocean to provide energy for life 7. Should this hypothesis hold true it would be possible for life forms to exist in the liquid phase of its oceans, which reach depths of up to 170 km 7. While extra-terrestrial life remains a hypothesis, isolation and culturing of organisms living in extreme conditions on earth has shown that these organisms are very sensitive to changes in their environment 8-12. For example, when an organism that is adapted to more “mild” (atmospheric pressure) conditions is subjected to the extreme condition (high pressure) it is unable - 3 - to grow. 8. This is because changes in pressure, much like changes in temperature, have deleterious effects on biomacromolecules, including changes in the fluidity of membranes, the dissociation of protein-ligand complexes, and denaturation of proteins 9. A response of a system to changes in pressure is described by the Le Chatelier’s principle which suggests that a when a system in equilibrium is perturbed, it will re-equilibrate to a new state which minimizes the effect of the perturbation. Thermodynamically this can be written as: ∂∆G ( ) = ∆VTot 1. ∂P T where G is the Gibbs energy and VTot is the volume changes, P is the pressure and T is the temperature. In the case of pressure unfolding of proteins, the system is the protein in an aqueous environment, the perturbation is the application of the pressure, and the equilibrium is between 13-15 folded and unfolded states. The majority of proteins have a negative VTot of unfolding , i.e. the volume of the unfolded state is smaller than the volume of the native state. Thus, the increase in pressure will lead to protein unfolding. Interestingly, there are examples of proteins that have a positive VTot which suggests that the folded state will become more stable at increased pressure 15. Based on these observations, one of the possible mechanisms of adaptation to high hydrostatic pressure is that proteins from organisms that live under elevated pressure evolved to have less negative, or even positive, volume changes upon unfolding,. This hypothesis has been tested in the present work. We have previously established a method to compute the ΔVTot for proteins using its three- dimensional structure 15,16. To better understand the molecular mechanisms of adaptation to high pressure, we have applied this formalism to ΔVTot analysis of the proteome of organisms living under diverse environmental conditions which include psychro-piezophiles (high-pressure, low- temperature adapted organisms), thermo-piezophiles (high-temperature, high-pressure adapted - 4 - organisms,) thermophiles (high-temperature adapted organisms), and psychrophiles (low- temperature adapted organisms). We also analyzed the ΔVTot on a protein-by-protein basis, by finding homologous matches across the four groups mentioned above, and compared them to the proteins from mesophilic organisms. Finally, we experimentally measured and computed ΔVTot for two protein families, acylphosphatase and thioredoxin, using representative structures from these classes of organisms. 2. Materials and Methods Obtaining and Preparing Structures from PDB Groups of Archaea and Prokarya belonging to psychrophiles, psychro-piezophiles, thermophiles, and thermo-piezophiles were identified that contained structures deposited to the Protein Data Bank (PDB). Effort was taken to ensure that a diverse group of organisms was represented in the study, thus both bacterial and archaeal species were included in the four groups. Some organisms such as Thermus thermophilus is over represented in the Protein Data Bank, having more than 10,000 deposited structures; in such cases, the organism was left out of the initial analysis. This was done to minimize bias towards any single organism. Only structures solved by X-ray crystallography were included into analysis. Structures were obtained using the RESTful web service of the PDB. An XML document containing the PDB Tree Entity ID for an organism, chain type containing only proteins (yes Protein, no DNA, no RNA, no RNA/DNA hybrids), and an X-ray resolution of < 2.7 Å was uploaded to http://www.rcsb.org/pdb/rest/search. The search results returned a list of PDB IDs matching the aforementioned criteria. All structures were analyzed as monomers. In cases of homomultimers, chain A was selected to further analysis. In cases of heteromultimers, the first - 5 - representative chain of each sequence was chosen to undergo further analysis. Any structures that were discontinuous due to missing amino acids were discarded. To ensure low redundancy in the analysis, proteins with multiple structures were identified and the longest, continuous, structure was chosen, the rest were discarded. Sequences containing modified or unnatural amino acids were discarded. The only exception was for sequences containing selenomethionine. The Modeller software package was used to model in missing sidechain atoms 17. Complete list of PDB id is provided. Generating the Native and Unfolded Sate Ensembles To generate the native state ensemble, a native-state, all-atom, explicit solvent MD was performed using GROMACS, as previously described 15,18. The CHARMM27 force field and the TIP3P water model were used to model the protein water system 19,20. The obtained crystal structures were solvated in a dodecahedron such that the all of the protein atoms were at least 10 Å away from the edges of the box. Charges on the protein were neutralized by the addition of 0.1 M of NaCl. An initial energy minimization steepest descent was performed for 1000 integration steps or until the maximum force < 1,000 kJ/mol/nm was reached. This was followed by a 200 ps of constant volume equilibration, then 100 ps of constant pressure equilibration. Lastly, a 50 ns production run was generated at a temperature of 300 K and 1 bar of pressure. Pressure was monitored using the Parinello-Rahman barostat, having a 2 ps relaxation time and a compressibility of 4.6·10-5atm-1 21. Temperature was monitored with V-rescale, having a relaxation time of 0.1 ps 18. High frequency bond vibrations were constrained using the LINCS and SETTLE algorithms 22. Electrostatic interactions were modelled using a smooth Particle Mesh Ewald (PME), with a PME order paramour of four, and a Fourier spacing of 0.16 23. At the end of the - 6 - production run, 50 structures were extracted from the production trajectory at a sampling frequency of one structure per nanosecond in order to obtain the native state ensemble. The unfolded state ensemble containing 1000 structures for each protein was generated using Trajectory Directed Ensemble Sampling (TraDES) 24. Secondary structure propensity was removed using the all-coil flag in TraDES software (-c T). The generated structures were subsequently energy minimized in implicit solvent as described above.
Details
-
File Typepdf
-
Upload Time-
-
Content LanguagesEnglish
-
Upload UserAnonymous/Not logged-in
-
File Pages27 Page
-
File Size-