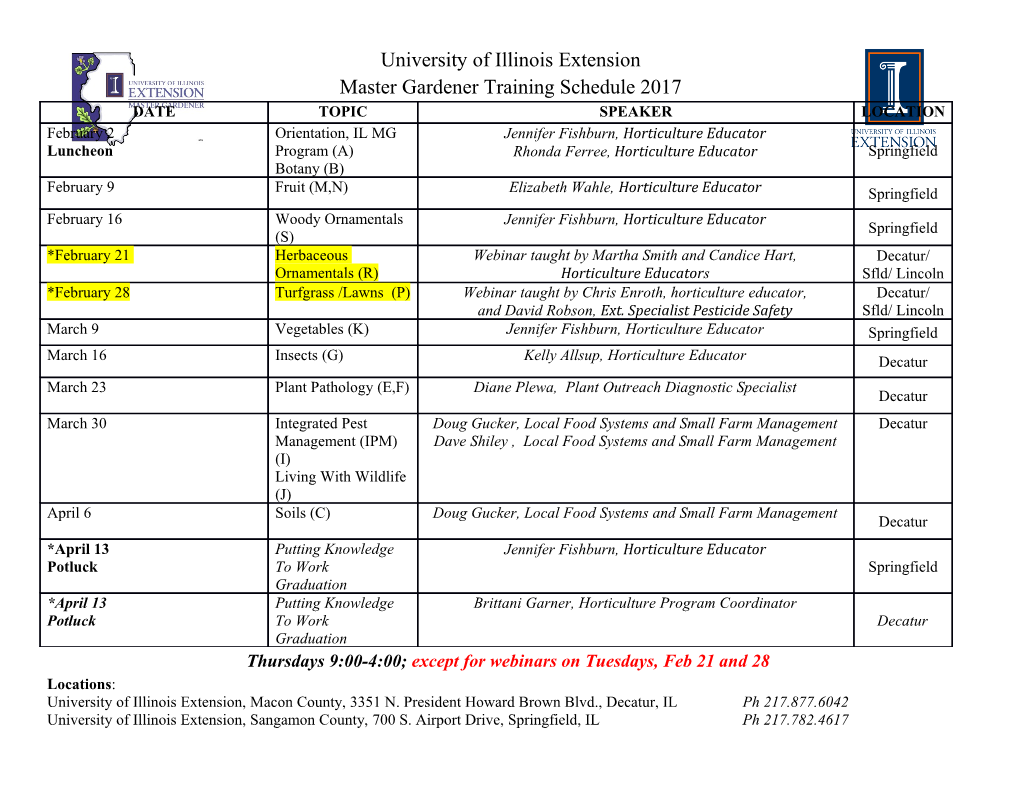
Selected for a Viewpoint in Physics week ending PRL 110, 153201 (2013) PHYSICAL REVIEW LETTERS 12 APRIL 2013 Spectroscopic Detection of the LiHe Molecule Naima Tariq, Nada Al Taisan, Vijay Singh, and Jonathan D. Weinstein* Department of Physics, University of Nevada, Reno, Nevada 89557, USA (Received 27 December 2012; published 8 April 2013) We use cryogenic helium buffer-gas cooling to form large densities of lithium atoms in a high-density helium gas, from which LiHe molecules form by three-body recombination. These weakly bound van der Waals molecules are detected spectroscopically, and their binding energy is measured from their equilibrium thermodynamic properties. DOI: 10.1103/PhysRevLett.110.153201 PACS numbers: 34.50.Lf, 33.15.Fm, 33.20.Àt van der Waals (vdW) molecules, which are bound by where n is the density of the given species, is the reduced long-range dispersion interactions, represent the most mass, T is the temperature, and gi and i are the degener- weakly bound form of molecular matter [1]. Of the dia- acies and binding energies of the LiHe bound states [16]. tomic vdW molecules, those containing helium—the most Clearly, the formation of detectable quantities of LiHe is chemically ‘‘inert’’ of the noble gases—are the most favored by high lithium and helium densities and low weakly bound. There is much theoretical interest in the temperatures. All three are achieved with cryogenic helium study of diatomic molecules involving helium, including buffer-gas cooling of atomic lithium produced by laser questions over their existence [2–4]. To date, the only ablation [17]. ground-state helium diatomic molecule that has been We note that the exceptionally low binding energy of directly detected in the gas phase is He2 [5–7]. lithium to helium makes the formation of LiHe less favor- Kleinekatho¨fer et al. [3] predict that for all 4He-alkali able than many other helium vdW molecules [18]. We diatomic molecules, there exists a single bound rovibra- chose to search for LiHe due to our group’s prior experi- tional state in the X2Æ ground electronic state. 7Li4He is ence working with atomic lithium [17], prior theoretical predicted to have a bond length of hri28 A , and a work on the structure of the LiHe molecule [3,19–22], and binding energy of 0:0039 cmÀ1 [3]. These numbers are because atomic lithium’s D transition is easily accessed 4 comparable to He2 [6], despite the significantly smaller with diode lasers. well depth of 7Li4He [8]. The experiment is conducted in a cryogenic cell, iden- Experimentally, vdW molecules have been investigated tical to the apparatus described in Ref. [17]. The cell through a variety of techniques. Supersonic expansions temperature is monitored by a ruthenium oxide resistor have produced argon- and neon-based vdW molecules for and a silicon diode. The helium density in the cell is spectroscopic studies as well as helium bound to large determined from a room-temperature pressure gauge con- molecules [9]. Excited-state helium vdW molecules have nected to the cell through a thin tube and from the cell been observed in liquid helium [10], in dense helium gas temperature. We correct for the thermomolecular pressure [11–13], and in superfluid helium nanodroplets [14,15]. ratio with the Weber-Schmidt equation [23]. The uncer- Brahms et al. observed that helium buffer-gas cooling tainty in the helium density is approximately Æ20%. would provide a favorable environment for forming Gas-phase Li is produced by laser ablation of a solid ground-state helium vdW molecules and demonstrated target of 99.9% pure Li (of natural isotopic abundances) indirect evidence for the formation of Ag3He [16]. with a frequency-doubled Nd:YAG laser. The Li atoms are Following this work, we have prepared high densities of detected by laser absorption spectroscopy on the D1 tran- cold lithium atoms in a cryogenic helium gas, in order to sition at 671 nm [24,25]. Due to the high atomic densities create LiHe molecules through three-body recombination, employed in the experiment, we typically measure the atomic lithium via the less-abundant 6Li isotope. The probe Li þ He þ He $ LiHe þ He: power is 1 W, with a beam diameter of a few mm. (At powers * 1 W, we observe optical pumping of the Li We have spectroscopically detected the LiHe molecules atoms, which makes accurate measurements of density produced, measured their equilibrium properties, and mea- difficult.) The helium buffer-gas density ranges from sured the binding energy of the ground state. 2 Â 1017 to 1 Â 1018 cmÀ3. In thermal equilibrium, the expected density of LiHe is We are able to produce Li densities up to 1011 cmÀ3, given by with ablation energies of tens of mJ. The ablation pulse momentarily increases the temperature of the gas [26], so h2 3=2X n ¼ n n g ei=kBT; (1) we directly measure the translational temperature of the LiHe Li He k T i 2 B i gas through spectroscopy of the atomic 6Li; under our 0031-9007=13=110(15)=153201(5) 153201-1 Ó 2013 American Physical Society week ending PRL 110, 153201 (2013) PHYSICAL REVIEW LETTERS 12 APRIL 2013 conditions, Doppler broadening is observed to be the We attribute the larger splitting to the excited-state struc- dominant broadening mechanism. ture and the smaller splitting of each pair to the hyperfine Typical Li lifetimes in the cell are on the order of 10À1 s. structure of the ground state, as shown in Fig. 2. We note that We note that the lithium density as a function of time is not the measured ground-state hyperfine splitting is within 2 described by simple exponential decay; this is commonly of the atomic 7Li ground-state hyperfine splitting. Due to seen in buffer-gas cooling experiments at high helium the extremely weak binding energy of the molecule, we density [27]. To avoid complications due to this complex would expect the hyperfine splitting to be unchanged at the behavior, we measure Li and LiHe simultaneously. level of accuracy of our measurement. From this hyperfine LiHe molecules are detected by laser induced fluores- splitting and the rotational structure of the excited state cence (LIF). The LiHe probe beam power is on the order of (discussed below), we identify the molecule as 7Li4He. a few mW, with a beam diameter of a few mm. We note that To verify the assignment of the transitions, we calcu- the LiHe probe beam is spatially overlapped with the Li lated the structure of the highest-lying bound vibrational probe beam to within 3 mm. This overlap is important, as level of the first excited state of LiHe. The molecular the density distribution of Li throughout the cell is potentials that correlate to the Heð1SÞLið2PÞ excited state expected to be nonuniform [17]. The LIF signal is observed are A2Å and B2Æ. We construct simple model potentials 2 2 using a Si photodiode. To calibrate the frequency of the for the A Å and B Æ states of LiHe using the Buckingham LiHe probe beam, part of the beam is split off and sent potential formula for each, through a 100 mm-long iodine cell at 300 K [28,29], and À C6 another beam is sent through a Fabry-Perot cavity so that ð Þ¼ C2r À V r C1e 6 : (2) the laser scan can be linearized. The LiHe LIF and the r iodine signals are shown in Fig. 1; the frequency is cali- The long-range coefficients C are taken from the brated from the iodine signal. We note that we see no 6 calculations of Zhu et al. [20], and C1 and C2 are evidence of pressure shifts (or pressure broadening) over determined by fitting to the potentials calculated by the density range explored in this experiment, to within our Behmenburg et al. [19]. À1 experimental error of 0:004 cm in absolute frequencies. We first performed single-channel calculations of the The spectrum is quite simple, due to the structure of the energy levels of the A2Å and B2Æ model potentials. No ground state of LiHe, which is predicted to have only a bound states were found for the B state, whereas the A state single bound rovibrational state [3]. As seen in Fig. 1, supported multiple vibrational levels. Unfortunately, these Æ we observe two pairs of lines separated by 0:1774 single-channel calculations (which omit mixing of the A À1 0:0025 cm . The splitting of each pair is the same to and B states and do not include the lambda doubling) do within our experimental error, with an average value of not reproduce the observed splitting to within the experi- À1 0:0276 Æ 0:0004 cm . mental error. 2.385 + 2.380 2.375 2.370 Iodine Signal + 2.5 A 2 + 2.0 -1 -1 -1 -1 1.5 1.0 14902.563 cm 14902.740 cm 14902.768 cm 14902.591 cm LiHe Fluorescence (V) 0.5 X 2 + 0.0 14902.5 14902.6 14902.7 14902.8 14902.9 FIG. 2 (color online). Schematic of the relevant LiHe energy Wavenumber (cm-1) levels, showing the ground-state hyperfine structure and the excited-state rotational structure and lambda doubling. Levels FIG. 1 (color online). Fluorescence spectrum of LiHe mole- are labeled þ and À according to their parity. The hyperfine cules. The LiHe fluorescence is plotted against the lower axis; the structure in the excited state is not shown, as it is not resolved in iodine signal used for frequency calibration is shown on the upper our spectra.
Details
-
File Typepdf
-
Upload Time-
-
Content LanguagesEnglish
-
Upload UserAnonymous/Not logged-in
-
File Pages5 Page
-
File Size-