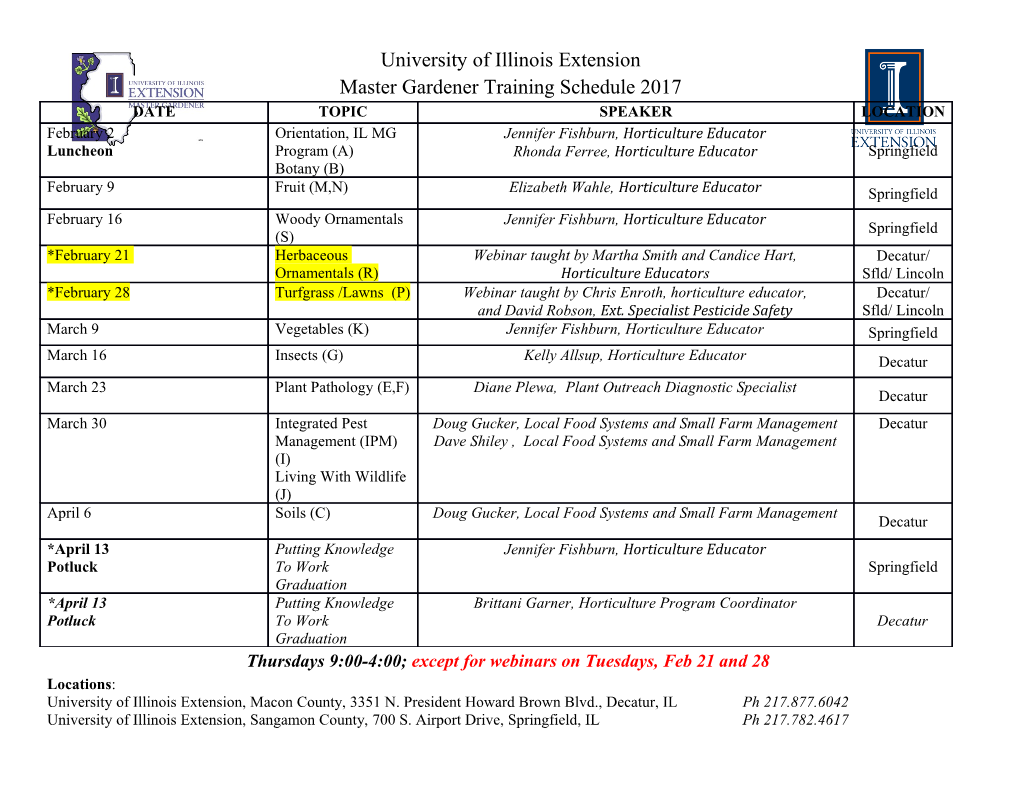
42nd Lunar and Planetary Science Conference (2011) 2340.pdf CONSTRAINTS ON THE STABILITIES OF OBSERVED MARTIAN SECONDARY MINERAL PHASES FROM GEOTHERMAL GRADIENT MODELS. B. C. Hahn1, H. Y. McSween1, and N. J. Tosca2, 1Department of Earth and Planetary Sciences, University of Tennessee, Knoxville ([email protected]), 2Department of Earth Sciences, University of Cambridge, Cambridge, UK. Introduction: The OMEGA and CRISM in- approximate crustal concentrations [13]. struments (aboard the Mars Express and the Mars Note that the GRS resolution is much larger than Observer orbiters, respectively) have detected a va- other remote sensing instruments and a single GRS riety of secondary mineral phases believed to have pixel is often significantly larger than an observed formed in the presence of water at specific, discrete feature. Therefore, calculated heat flows are repre- locations on the Martian surface [1, 2]. These detec- sentative of the general, not specific, areas observed. tions have included various hydrated silicate [3], car- Despite a penetration depth of only a few decimeters bonate [4], and sulfate mineral deposits [5]. Many of [15], at GRS resolution, global surface averages of K these detections are of secondary mineral rich layers and Th are believed to be broadly representative of exhumed from or exposed at some depth [6, 7]. Here the bulk Martian crust. It is estimated that 50% of we attempt to place thermal constraints on the stabili- Martian HPE budget has been sequestered into the ty of these secondary mineral phases (as reported by crust [16] and therefore calculated Martian crustal [8]) based on heat flow and geothermal gradients heat flow values represent half of the total present- modeled at the time of mineral formation and burial. day planetary thermal budget. Background: Previous studies have noted in de- Geothermal gradients are calculated following tail that many of these minerals do not persist for the method detailed in [17]. Heat flow and geother- long after deposition due to diagenetic processes re- mal models are then back-calculated to provide val- lated to burial, thermal constraints, and the presence ues for the time of formation or burial and for ancient and duration of available water [8]. For example, geologic surfaces and terrains; temperatures can be Tosca and Knoll (2009) report that hydrated amorph- much higher than present day values [13]. For exam- ous silica deposits (opaline), such as those analyzed ple, Figure 1 plots a typical terrestrial present day by Milliken et al. (2008) [9], is a juvenile chemical geothermal gradient for continental crust versus a sediment that eventually and rapidly transforms di- comparable average Martian gradient calculated for agenetically into microcrystalline quartz in the pres- the Late Noachian. In terrestrial continental crust, ence of heat and water; suggesting a water-limited HPE concentrations decrease exponentially with Martian environment [8, 10]. Likewise, as also re- depth due to granitoid-forming intra-crustal melting ported in [8], deposits of smectite clays [3, 11] rapid- and tectonic processes. However, only small regions ly convert into more stable illite or chlorite after of granitoid composition have been detected [18] and burial diagenesis [12]. As there is a strong depen- these processes are not apparent over large scales on dence on temperature for the conversion of these and Mars. Therefore, these models assume a vertically similar phases, it is important to constrain the thermal regime of these mineral deposits. However, until re- cently, geothermal gradients could only be calculated for certain geologic features using indirect geophysi- cal methods. excavated sediments? Methods: More recently, geothermal gradients can be modeled based on crustal heat flow estimates derived from heat-producing element (HPE) concen- trations (i.e., K, Th, and U) directly measured by the Gamma-Ray Spectrometer (GRS) onboard the Mars Odyssey orbiter [13]. The GRS instrument has mapped K and Th concentrations at a 5°x5° per pixel global resolution. While GRS can detect U, surface concentrations are too low for mapping at a similar resolution. However, U can be estimated assuming a Figure 1: Simplified representative geothermal gradient for an average Late Noachian Mars compared to ratio of Th/U = 3.8; a canonical cosmochemical value present day terrestrial continental crust [17]. Observed thought to be representative of most planetary bodies sediments have been buried to depths of at least a few and which also agrees with analyses of most martian kilometers. Average crustal radiogenic heat production meteorites [14]. HPE concentrations are also renor- during the Noachian is approximately 5 times that of malized to a S-, Cl-, and H2O-free basis to better present day [13]. 42nd Lunar and Planetary Science Conference (2011) 2340.pdf homogeneous HPE distribution throughout the crust and a constant crustal density of 3300 kg·m-3 for Nili Fossae/Baldet Crater these calculations. We also assume an average ther- mal conductivity of ~3 W·m-1·K-1; however, this can vary for regions exhibiting unusual primary silicate mineralogies significantly different from the global mean (as determined by TES and THEMIS mea- surements). Results & Discussion: While this abstract has insufficient space to discuss all the many instances of reported buried chemical sediments, as examples K: 3010 ppm here we report calculations for two such areas of re- Th: 0.69 ppm cent special importance. We will further explore U: ~0.18 ppm these and several other examples in more detail. Q: 50 mW∙m‐2 Of particular interest are regions exhibiting evi- 12.5 °C/km dence of past water activity that serve as possible landing site locations for the Mars Science Laborato- Figure 3: Measured HPE concentrations and calcu- ry (MSL). For example, Milliken et al. (2010) re- lated heat flow and geothermal gradient for the Nili Fos- ported detections of deeply buried clay-bearing lay- sae region during the Noachian; location of detected ers in Gale Crater (from depths of at least ~5 km) [7]. exposed buried phyllosilicates, hydrated silica, and car- Figure 2 shows the renormalized HPE abundances, bonates [4]. heat flow, and geothermal gradients for this general several crater walls) [4]. Figure 3 shows the renor- region as calculated for the Late Noachian-Early malized HPE abundances, heat flow, and geothermal Hesperian. Given a gradient of approximately 15 gradients for this general region as calculated for the °C/km and the observed burial depths, this implies a Noachian. Hydrated silica rapidly converts entirely to minimum temperature at depth of ~75 °C. As per the opal and then quartz over very short timescales in the models reported in [8], at this temperature, in the presence of liquid water and under moderate temper- presence of liquid water, smectite would rapidly con- atures. Given a HPE calculated gradient for the Nili vert almost entirely to illite (<100 Myr). Therefore, Fossae region of approximately 12.5 °C/km and the the prominent detection of persistent smectite at this observed burial depths of order >1 km (the highly location may imply limited aqueous interactions. eroded terrain suggests this is a minimum), this im- Similarly, Ehlmann et al. (2008) reported detec- plies a minimum temperature at depth of >12.5 °C. tion of a variety of minerals in the Nili Fossae region Again, using these calculations and the methods and (smectite clays, carbonates and hydrated silica within models of [8], at this temperature, hydrated silica would rapidly convert to below detection, while Gale Crater quartz has not been detected – again suggesting mi- nimal aqueous interaction. We will also report on calculated heat flow con- straints with respect to other locations and minerals, such as sulfates and carbonates. References: [1] Bibring J.-P. et al. (2005) Science, 307, 1576-1581. [2] Murchie S. L. et al. (2009) JGR, E00D07. [3] Poulet F., et al. (2005) Nature, 438, 623-627. [4] Ehlmann B. L. et al. (2009) JGR, E00D08. [5] Lange- vin Y. et al. (2005) Science, 307, 1584-1586. [6] Murchie S. L. (2009) JGR, E00D05. [7] Milliken R. E. et al. (2010) K: 3920 ppm GRL, L04201. [8] Tosca N. J. and Knoll A. H. (2009) Th: 0.74 ppm U: ~0.2 ppm EPSL, 286, 379-386. [9] Milliken R. E. et al. (2008) Geol- Q: ~60 mW∙m‐2 ogy, 36, 847-850. [10] McLennan S. M. (2003) Geology, 15 °C/km 31, 315-318. [11] Bishop J. L. et al. (2008) Science, 321, 830-833. [12] Weaver C. E. (1989) Clays, Muds, and Shales, Elsevier, NY. [13] Hahn B. C. et al. (2010) GRL, Figure 2: Measured HPE concentrations and calcu- submitted. [14] Meyer C. (2003) Mars Meteorite Compen- lated heat flow (Q) and geothermal gradient for the dium. [15] Boynton W. V. et al. (2007) JGR, 112, E12S99. Gale Crater region during the Late Noachian-Early [16] Taylor G. J. et al. (2006) JGR, 111, E03S06. [17] Tur- Hesperian; detection location of excavated deeply bu- cotte D. L. and Schubert G. (2002) Geodynamics, Cam- ried clay-bearing layers and a possible future MSL bridge University Press, UK. [18] Bandfield J. L. (2006) landing site [7]. GRL, L06203. .
Details
-
File Typepdf
-
Upload Time-
-
Content LanguagesEnglish
-
Upload UserAnonymous/Not logged-in
-
File Pages2 Page
-
File Size-