Ecological Interactions Between Euphydryas Editha Larvae and Their Host Plants
Total Page:16
File Type:pdf, Size:1020Kb
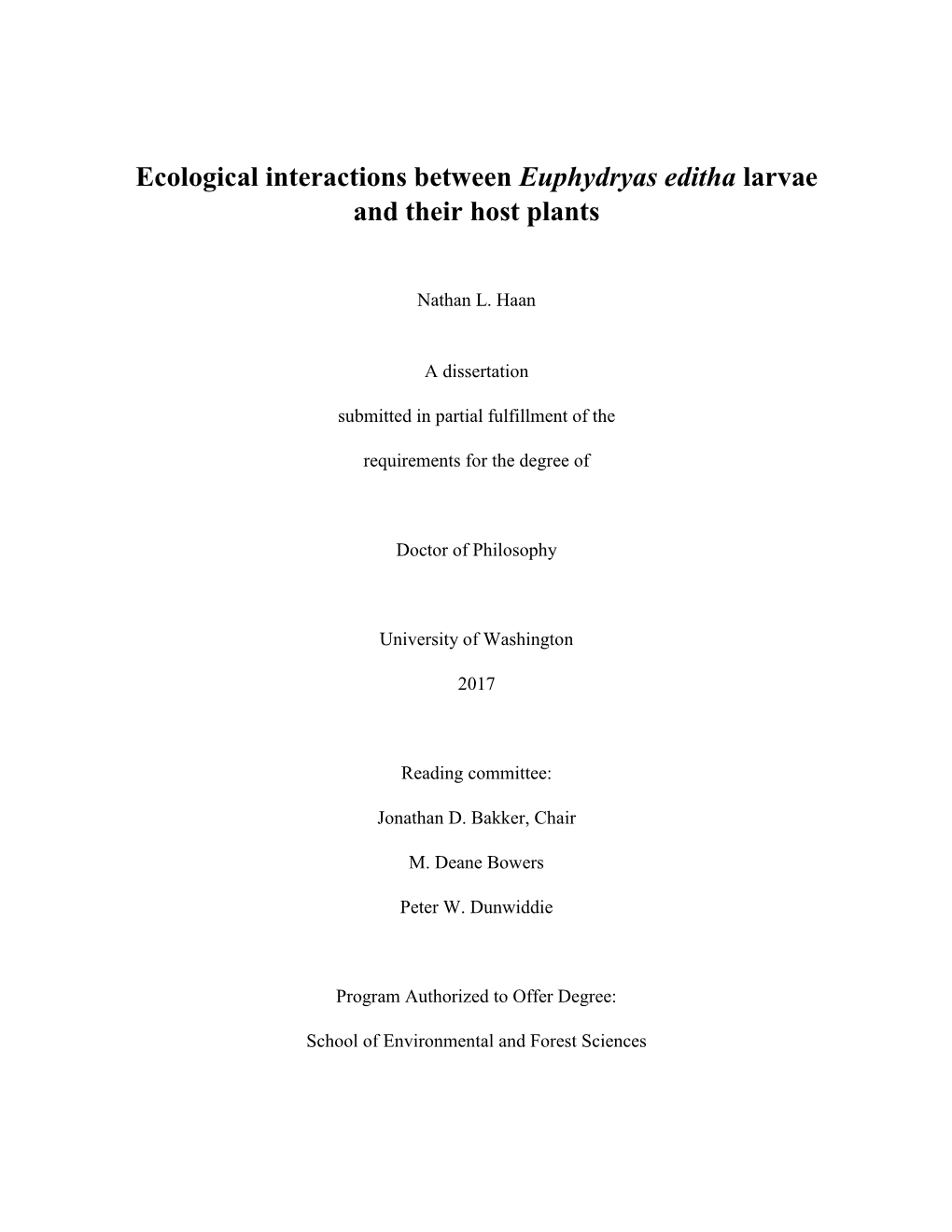
Load more
Recommended publications
-
Deraeocoris Schach, a New Predator of Euphydryas Aurinia and Other Heteropteran Feeding Habits on Caterpillar Web (Heteroptera: Miridae; Lepidoptera: Nymphalidae)
Fragmenta entomologica, 48 (1): 77-81 (2016) eISSN: 2284-4880 (online version) pISSN: 0429-288X (print version) Research article Submitted: March 21st, 2016 - Accepted: June 10th, 2016 - Published: June 30th, 2016 Deraeocoris schach, a new predator of Euphydryas aurinia and other heteropteran feeding habits on caterpillar web (Heteroptera: Miridae; Lepidoptera: Nymphalidae) Manuela PINZARI Dipartimento di Biologia, Università di Roma Tor Vergata - Via della Ricerca Scientifica 1, I-00133 Roma, Italy [email protected] Abstract In this paper, preliminary results on a field study aiming to identify predators of the Marsh Fritillary Euphydryas aurinia (Rottemburg, 1775) in Central Italy are presented. Several heteropterans were found on the larval nests of E. aurinia for dietary reasons: Deraeoco ris schach (Fabricius, 1781) that is a predator of Marsh Fritillary larvae, Palomena prasina (Linnaeus, 1761) and Spilostethus saxati lis (Scopoli, 1763) that feed on the droppings of larvae; Graphosoma lineatum italicum (Müller, 1766) that visits the larval web during winter diapause. Key words: Euphydryas aurinia, Deraeocoris schach, predator, heteropterans. Introduction Schult, Lonicera caprifolium L. and Scabiosa columbar ia L. (Pinzari, Pinzari and Sbordoni, unpublished data), In the context of a previous survey study (Pinzari et al. with spiders and different insects (Orthoptera, Heterop- 2010, 2013) on the Lepidoptera in Central Apennines tera, Diptera, Hymenoptera and Blattellidae) that usually (Lazio, Italy), during the past five years a population of frequent the larval nests of E. aurinia. E. aurinia spp. provincialis (Boisduval, 1828) was stud- In literature predation by Heteroptera has been ob- ied, focusing on several aspects of the species biology and served in the American Checkerspots, E. -
Conceptual Design Documentation
Appendix A: Conceptual Design Documentation APPENDIX A Conceptual Design Documentation June 2019 A-1 APPENDIX A: CONCEPTUAL DESIGN DOCUMENTATION The environmental analyses in the NEPA and CEQA documents for the proposed improvements at Oceano County Airport (the Airport) are based on conceptual designs prepared to provide a realistic basis for assessing their environmental consequences. 1. Widen runway from 50 to 60 feet 2. Widen Taxiways A, A-1, A-2, A-3, and A-4 from 20 to 25 feet 3. Relocate segmented circle and wind cone 4. Installation of taxiway edge lighting 5. Installation of hold position signage 6. Installation of a new electrical vault and connections 7. Installation of a pollution control facility (wash rack) CIVIL ENGINEERING CALCULATIONS The purpose of this conceptual design effort is to identify the amount of impervious surface, grading (cut and fill) and drainage implications of the projects identified above. The conceptual design calculations detailed in the following figures indicate that Projects 1 and 2, widening the runways and taxiways would increase the total amount of impervious surface on the Airport by 32,016 square feet, or 0.73 acres; a 6.6 percent increase in the Airport’s impervious surface area. Drainage patterns would remain the same as both the runway and taxiways would continue to sheet flow from their centerlines to the edge of pavement and then into open, grassed areas. The existing drainage system is able to accommodate the modest increase in stormwater runoff that would occur, particularly as soil conditions on the Airport are conducive to infiltration. Figure A-1 shows the locations of the seven projects incorporated in the Proposed Action. -
Lepidoptera Recorded for Imperial County California Compiled by Jeffrey Caldwell [email protected] 1-925-949-8696 Note
Lepidoptera Recorded for Imperial County California Compiled by Jeffrey Caldwell [email protected] 1-925-949-8696 Note: BMNA = Butterflies and Moths of North America web site MPG = Moth Photographers Group web site Most are from the Essig Museum’s California Moth Specimens Database web site Arctiidae. Tiger and Lichen Moths. Apantesis proxima (Notarctia proxima). Mexican Tiger Moth. 8181 [BMNA] Ectypia clio (clio). Clio Tiger Moth. 8249 Estigmene acrea (acrea). Salt Marsh Moth. 8131 Euchaetes zella. 8232 Autostichidae (Deoclonidae). Oegoconia novimundi. Four-spotted Yellowneck Moth. 1134 (Oegoconia quadripuncta mis-applied) Bucculatricidae. Ribbed Cocoon-maker Moths. Bucculatrix enceliae. Brittlebrush Moth. 0546 Cossidae. Goat Moths, Carpenterworm Moths, and Leopard Moths. Comadia henrici. 2679 Givira mucida. 2660 Hypopta palmata. 2656 Prionoxystus robiniae (mixtus). Carpenterworm or Locust Borer. 2693 Depressariidae. Pseudethmia protuberans. 1008 [MPG] Ethmiidae. Now assigned to Depressariidae. Ethmiinae. Ethmia timberlakei. 0984 Pseudethmia protuberans. 1008 Gelechiidae. Twirler Moths. Aristotelia adceanotha. 1726 [Sighting 1019513 BMNA] Chionodes abdominella. 2054 Chionodes dentella. 2071 Chionodes fructuaria. 2078 Chionodes kincaidella. 2086 (reared from Atriplex acanthocarpa in Texas) Chionodes oecus. 2086.2 Chionodes sistrella. 2116 Chionodes xanthophilella. 2125 Faculta inaequalis. Palo Verde Webworm. 2206 Friseria cockerelli. Mesquite Webworm. 1916 Gelechia desiliens. 1938 Isophrictis sabulella. 1701 Keiferia lycopersicella. Tomato Pinworm. 2047 Pectinophora gossypiella. Pink Bollworm. 2261 Prolita puertella. 1895 Prolita veledae. 1903 Geometridae. Inchworm Moths, Loopers, Geometers, or Measuring Worms. Archirhoe neomexicana. 7295 Chesiadodes coniferaria. 6535 Chlorochlamys appellaria. 7073 Cyclophora nanaria. Dwarf Tawny Wave. W 7140 Dichorda illustraria. 7055 Dichordophora phoenix. Phoenix Emerald. 7057 Digrammia colorata. Creosote Moth. 6381 Digrammia irrorata (rubricata). 6395 Digrammia pictipennata. 6372 Digrammia puertata. -
Host Plants and Habitats of the Baltimore Checkerspot Butterfly, Euphydryas Phaeton (Lepidoptera: Nymphalidae), in the Great Lakes Region
The Great Lakes Entomologist Volume 24 Number 4 - Winter 1991 Number 4 - Winter Article 1 1991 December 1991 Host Plants and Habitats of the Baltimore Checkerspot Butterfly, Euphydryas Phaeton (Lepidoptera: Nymphalidae), in the Great Lakes Region Brian G. Scholtens University of Michigan Follow this and additional works at: https://scholar.valpo.edu/tgle Part of the Entomology Commons Recommended Citation Scholtens, Brian G. 1991. "Host Plants and Habitats of the Baltimore Checkerspot Butterfly, Euphydryas Phaeton (Lepidoptera: Nymphalidae), in the Great Lakes Region," The Great Lakes Entomologist, vol 24 (4) Available at: https://scholar.valpo.edu/tgle/vol24/iss4/1 This Peer-Review Article is brought to you for free and open access by the Department of Biology at ValpoScholar. It has been accepted for inclusion in The Great Lakes Entomologist by an authorized administrator of ValpoScholar. For more information, please contact a ValpoScholar staff member at [email protected]. Scholtens: Host Plants and Habitats of the Baltimore Checkerspot Butterfly, 1991 THE GREAT LAKES ENTOMOLOGIST 207 HOST PLANTS AND HABITATS OF THE BALTIMORE CHECKERSPOT BUTTERFLY, EUPHYDRYAS PHAETON (LEPIDOPTERA: NYMPHALIDAE), IN THE GREAT LAKES REGION Brian G. Scholtens 1 ABSTRACT The habitats and host plants of Euphydryas phaeton in the Great Lakes region are examined using data from several different populations spread over much of the region. The range of habitats and host plants used by this species is wider than commonly believed. While many populations are found in seasonal or permanent wetlands, others are located in dry, old fields or woodland areas. The host plants used vary with habitat, but they include all major primary hosts and many second ary hosts previously reported plus several new records. -
2010 Season Summary Index NEW WOFTHE~ Zone 1: Yukon Territory
2010 Season Summary Index NEW WOFTHE~ Zone 1: Yukon Territory ........................................................................................... 3 Alaska ... ........................................ ............................................................... 3 LEPIDOPTERISTS Zone 2: British Columbia .................................................... ........................ ............ 6 Idaho .. ... ....................................... ................................................................ 6 Oregon ........ ... .... ........................ .. .. ............................................................ 10 SOCIETY Volume 53 Supplement Sl Washington ................................................................................................ 14 Zone 3: Arizona ............................................................ .................................... ...... 19 The Lepidopterists' Society is a non-profo California ............... ................................................. .............. .. ................... 2 2 educational and scientific organization. The Nevada ..................................................................... ................................ 28 object of the Society, which was formed in Zone 4: Colorado ................................ ... ............... ... ...... ......................................... 2 9 May 1947 and formally constituted in De Montana .................................................................................................... 51 cember -
Euphydryas Gillettii (Nymphalidae)
Journal of the Lepidopterists' Society 42(1), 1988, 37-45 HABIT A T AND RANGE OF EUPHYDRYAS GILLETTII (NYMPHALIDAE) ERNEST H. WILLIAMS Department of Biology, Hamilton College, Clinton, New York 13323 ABSTRACT. Fifteen sites occupied by Euphydryas gillettii are compared according to 10 characteristics. All sites are moist, open, mostly montane meadows, many with a history of disturbance, commonly fire. Population size correlates with relative availability of nectar but not with overall abundance of the usual hostplant, Lonicera involucrata. Habitats at higher latitudes often have a southerly exposure. Reduction in hostplant size at higher latitudes contributes to the northern range limit. Three populations likely have become extinct since 1960, but the species range does not appear to be changing. Additional key words: nectar, Lonicera involucrata, biogeography, extinction. Euphydryas gillettii (Barnes), a checkers pot butterfly, occurs in dis crete, isolated populations (Williams et al. 1984) in the central and northern Rocky Mountains (Ferris & Brown 1981). It is attractive and easily caught but uncommon and not often collected. Though usually considered a montane species (Williams et al. 1984), variation in sites occupied by E. gillettii has not been studied, and lack of knowledge about its habitats has led to uncertainty about its range. Here I report characteristics of sites occupied by E. gillettii, present range of the species, and factors influencing its distributional pattern. This study is based on direct observation of the habitats of 15 populations throughout the range, thus affording an uncommon view of habitat variability in a single insect species. METHODS Populations of E. gillettii were located through correspondence with '. -
Gillette's Checkerspot Euphydryas Gillettii
Gillette’s Checkerspot Euphydryas gillettii Insecta — Lepidoptera — Nymphalidae CONSERVATION STATUS / CLASSIFICATION Rangewide: Imperiled/Vulnerable (G2G3) Statewide: Vulnerable (S3) ESA: No status USFS: Region 1: No status; Region 4: No status BLM: No status IDFG: Not classified BASIS FOR INCLUSION Lack of essential information pertaining to status; no trend data. TAXONOMY Euphydryas gillettii was described in 1897 from specimens collected in Yellowstone National Park (Williams et al. 1984). DISTRIBUTION AND ABUNDANCE This checkerspot occurs in discrete, isolated populations across the montane parts of Idaho, Montana, Wyoming, Alberta, and British Columbia. In Idaho, this species has been reported to occur in scattered localities throughout the central and eastern mountains. Individual populations are typically small, often comprising <30 adults, and widely separated from neighboring populations (Debinski 1994). Populations are biennial and can fluctuate greatly in abundance from year to year (Williams 1988; Williams et al. 1984). POPULATION TREND Population trend is unknown. HABITAT AND ECOLOGY This species is associated with open and sunny clearings in mesic habitat, often occurring near small streams or in marshy habitat. Many colonies occur at sites where fire-disturbed sites where the butterfly’s host plant and nectar sources occur in openings in forested habitat created by fire (Williams 1988). Twinberry honeysuckle (Lonicera involucrata) is the primary larval host plant, and western valeriana (Valeriana occidentalis), lousewort (Pedicularis sp.), speedwell (Veronica wormskjoldii), and snowberry (Symphoricarpus albus) are also known to be host plants (Williams 1988). Abundance at a given site is correlated with the abundance of nectar sources. Williams (1988) commented that “life in disturbed sites suggests that E. -
State Status Federal Status Scientific Name/Common Name Element
California Department of Fish and Game Natural Diversity Database Selected Elements by Scientific Name - Portrait Grover Beach Lodge RareFind List 7-27-2010; Oceano, Nipomo, Point Sal, Guadalupe, Santa Maria, Pismo Beach, Arroyo Grande NE, Tar Spring Ridge CDFG or Scientific Name/Common Name Element Code Federal Status State Status GRank SRank CNPS 1 Ablautus schlingeri IIDIP42010 G1 S1 Oso Flaco robber fly 2 Accipiter striatus ABNKC12020 G5 S3 sharp-shinned hawk 3 Agrostis hooveri PMPOA040M0 G2 S2.2 1B.2 Hoover's bent grass 4 Ambystoma californiense AAAAA01180 Threatened Threatened G2G3 S2S3 SC California tiger salamander 5 Anniella pulchra pulchra ARACC01012 G3G4T3T4 S3 SC silvery legless lizard Q 6 Aphanisma blitoides PDCHE02010 G2 S1.1 1B.2 aphanisma 7 Arctostaphylos luciana PDERI040N0 G2 S2.2 1B.2 Santa Lucia manzanita 8 Arctostaphylos morroensis PDERI040S0 Threatened G2 S2.2 1B.1 Morro manzanita 9 Arctostaphylos pechoensis PDERI04140 G2 S2.2 1B.2 Pecho manzanita 10 Arctostaphylos pilosula PDERI04160 G2 S2.2 1B.2 Santa Margarita manzanita 11 Arctostaphylos rudis PDERI041E0 G2 S2.2 1B.2 sand mesa manzanita 12 Arctostaphylos wellsii PDERI042B0 G1G2 S1S2 1B.1 Wells' manzanita 13 Arenaria paludicola PDCAR040L0 Endangered Endangered G1 S1 1B.1 marsh sandwort 14 Areniscythris brachypteris IILEG49010 G1 S1 Oso Flaco flightless moth 15 Astragalus didymocarpus var. milesianus PDFAB0F2X3 G5T2 S2.2 1B.2 Miles' milk-vetch 16 Athene cunicularia ABNSB10010 G4 S2 SC burrowing owl 17 Atriplex serenana var. davidsonii PDCHE041T1 G5T2? S2? 1B.2 Davidson's saltscale 18 Branchinecta lynchi ICBRA03030 Threatened G3 S2S3 vernal pool fairy shrimp 19 Calochortus obispoensis PMLIL0D110 G2 S2.1 1B.2 La Panza mariposa-lily 20 Calochortus simulans PMLIL0D170 G2 S2.3 1B.3 San Luis Obispo mariposa-lily 21 Calystegia subacaulis ssp. -
Quino Checkerspot Butterfly Survey Report 2017
Western Riverside County Multiple Species Habitat Conservation Plan Biological Monitoring Program 2017 Quino Checkerspot Butterfly (Euphydryas editha quino) Survey Report 22 June 2018 2017 Quino Checkerspot Butterfly Survey Report TABLE OF CONTENTS INTRODUCTION .................................................................................................................... 1 GOALS AND OBJECTIVES ................................................................................................................... 2 METHODS ............................................................................................................................ 3 PROTOCOL DEVELOPMENT ................................................................................................................ 3 STUDY SITE SELECTION ..................................................................................................................... 3 SURVEY METHODS ............................................................................................................................ 6 TRAINING ........................................................................................................................................... 7 DATA ANALYSIS ................................................................................................................................ 7 RESULTS .............................................................................................................................. 8 SENTINEL SITE SURVEYS .................................................................................................................. -
Papilio (New Series) # 25 2016 Issn 2372-9449
PAPILIO (NEW SERIES) # 25 2016 ISSN 2372-9449 ERNEST J. OSLAR, 1858-1944: HIS TRAVEL AND COLLECTION ITINERARY, AND HIS BUTTERFLIES by James A. Scott, Ph.D. in entomology University of California Berkeley, 1972 (e-mail: [email protected]) Abstract. Ernest John Oslar collected more than 50,000 butterflies and moths and other insects and sold them to many taxonomists and museums throughout the world. This paper attempts to determine his travels in America to collect those specimens, by using data from labeled specimens (most in his remaining collection but some from published papers) plus information from correspondence etc. and a few small field diaries preserved by his descendants. The butterfly specimens and their localities/dates in his collection in the C. P. Gillette Museum (Colorado State University, Fort Collins, Colorado) are detailed. This information will help determine the possible collection locations of Oslar specimens that lack accurate collection data. Many more biographical details of Oslar are revealed, and the 26 insects named for Oslar are detailed. Introduction The last collection of Ernest J. Oslar, ~2159 papered butterfly specimens and several moths, was found in the C. P. Gillette Museum, Colorado State University, Fort Collins, Colorado by Paul A. Opler, providing the opportunity to study his travels and collections. Scott & Fisher (2014) documented specimens sent by Ernest J. Oslar of about 100 Argynnis (Speyeria) nokomis nokomis Edwards labeled from the San Juan Mts. and Hall Valley of Colorado, which were collected by Wilmatte Cockerell at Beulah New Mexico, and documented Oslar’s specimens of Oeneis alberta oslari Skinner labeled from Deer Creek Canyon, [Jefferson County] Colorado, September 25, 1909, which were collected in South Park, Park Co. -
Nr 222 Native Tree, Shrub, & Herbaceous Plant
NR 222 NATIVE TREE, SHRUB, & HERBACEOUS PLANT IDENTIFICATION BY RONALD L. ALVES FALL 2014 NR 222 by Ronald L. Alves Note to Students NOTE TO STUDENTS: THIS DOCUMENT IS INCOMPLETE WITH OMISSIONS, ERRORS, AND OTHER ITEMS OF INCOMPETANCY. AS YOU MAKE USE OF IT NOTE THESE TRANSGRESSIONS SO THAT THEY MAY BE CORRECTED AND YOU WILL RECEIVE A CLEAN COPY BY THE END OF TIME OR THE SEMESTER, WHICHEVER COMES FIRST!! THANKING YOU FOR ANY ASSISTANCE THAT YOU MAY GIVE, RON ALVES. Introduction This manual was initially created by Harold Whaley an MJC Agriculture and Natural Resources instruction from 1964 – 1992. The manual was designed as a resource for a native tree and shrub identification course, Natural Resources 222 that was one of the required courses for all forestry and natural resource majors at the college. The course and the supporting manual were aimed almost exclusively for forestry and related majors. In addition to NR 222 being taught by professor Whaley, it has also been taught by Homer Bowen (MJC 19xx -), Marlies Boyd (MJC 199X – present), Richard Nimphius (MJC 1980 – 2006) and currently Ron Alves (MJC 1974 – 2004). Each instructor put their own particular emphasis and style on the course but it was always oriented toward forestry students until 2006. The lack of forestry majors as a result of the Agriculture Department not having a full time forestry instructor to recruit students and articulate with industry has resulted in a transformation of the NR 222 course. The clientele not only includes forestry major, but also landscape designers, environmental horticulture majors, nursery people, environmental science majors, and people interested in transforming their home and business landscapes to a more natural venue. -
A SKELETON CHECKLIST of the BUTTERFLIES of the UNITED STATES and CANADA Preparatory to Publication of the Catalogue Jonathan P
A SKELETON CHECKLIST OF THE BUTTERFLIES OF THE UNITED STATES AND CANADA Preparatory to publication of the Catalogue © Jonathan P. Pelham August 2006 Superfamily HESPERIOIDEA Latreille, 1809 Family Hesperiidae Latreille, 1809 Subfamily Eudaminae Mabille, 1877 PHOCIDES Hübner, [1819] = Erycides Hübner, [1819] = Dysenius Scudder, 1872 *1. Phocides pigmalion (Cramer, 1779) = tenuistriga Mabille & Boullet, 1912 a. Phocides pigmalion okeechobee (Worthington, 1881) 2. Phocides belus (Godman and Salvin, 1890) *3. Phocides polybius (Fabricius, 1793) =‡palemon (Cramer, 1777) Homonym = cruentus Hübner, [1819] = palaemonides Röber, 1925 = ab. ‡"gunderi" R. C. Williams & Bell, 1931 a. Phocides polybius lilea (Reakirt, [1867]) = albicilla (Herrich-Schäffer, 1869) = socius (Butler & Druce, 1872) =‡cruentus (Scudder, 1872) Homonym = sanguinea (Scudder, 1872) = imbreus (Plötz, 1879) = spurius (Mabille, 1880) = decolor (Mabille, 1880) = albiciliata Röber, 1925 PROTEIDES Hübner, [1819] = Dicranaspis Mabille, [1879] 4. Proteides mercurius (Fabricius, 1787) a. Proteides mercurius mercurius (Fabricius, 1787) =‡idas (Cramer, 1779) Homonym b. Proteides mercurius sanantonio (Lucas, 1857) EPARGYREUS Hübner, [1819] = Eridamus Burmeister, 1875 5. Epargyreus zestos (Geyer, 1832) a. Epargyreus zestos zestos (Geyer, 1832) = oberon (Worthington, 1881) = arsaces Mabille, 1903 6. Epargyreus clarus (Cramer, 1775) a. Epargyreus clarus clarus (Cramer, 1775) =‡tityrus (Fabricius, 1775) Homonym = argentosus Hayward, 1933 = argenteola (Matsumura, 1940) = ab. ‡"obliteratus"