The Dusty High Redshift Universe: the Dust Content and Its Effects in the first Galaxies
Total Page:16
File Type:pdf, Size:1020Kb
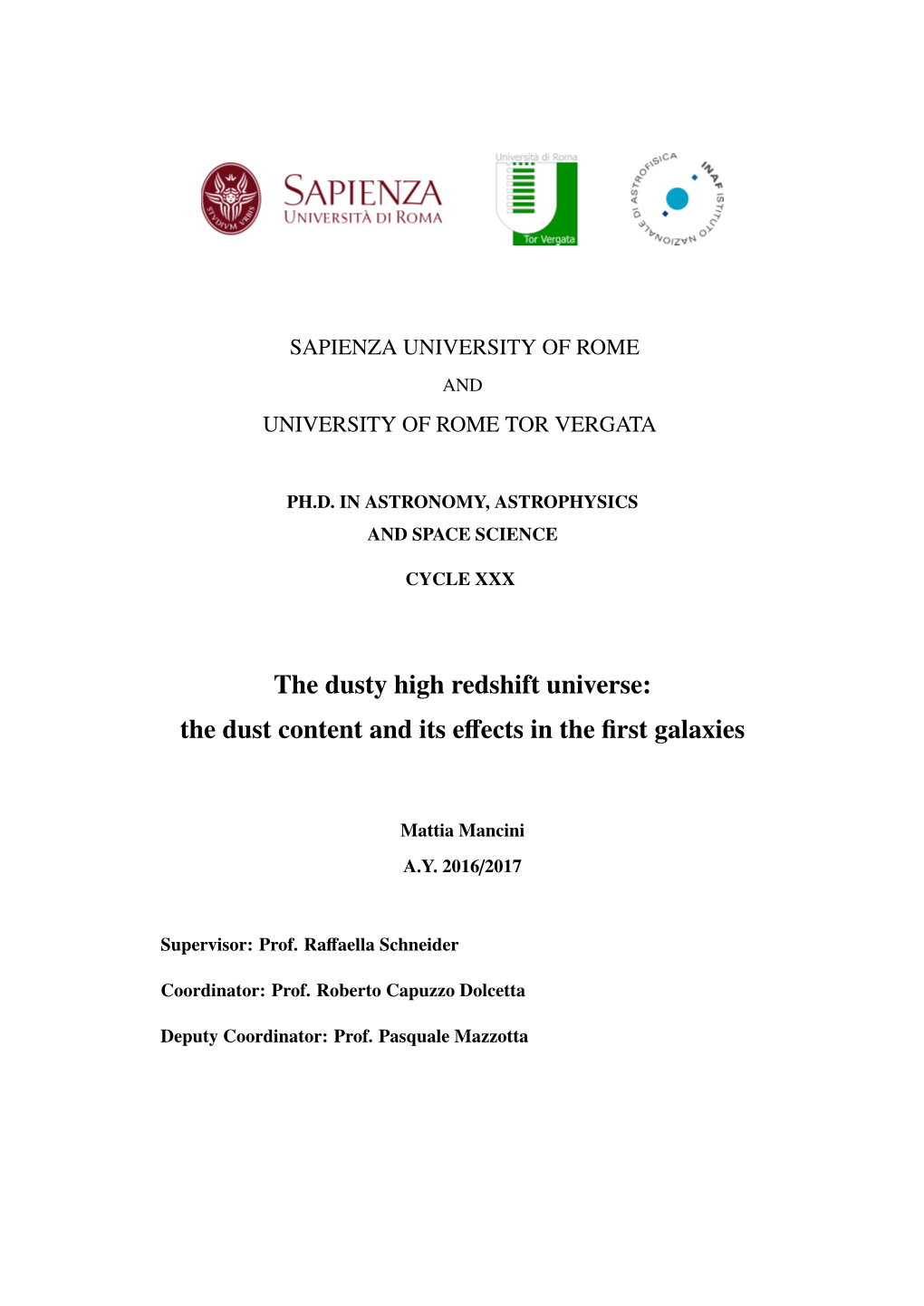
Load more
Recommended publications
-
A Dusty, Normal Galaxy in the Epoch of Reionization
A dusty, normal galaxy in the epoch of reionization Darach Watson1, Lise Christensen1, Kirsten Kraiberg Knudsen2, Johan Richard3, Anna Gallazzi4,1, and Michał Jerzy Michałowski5 Candidates for the modest galaxies that formed most of the stars in the early universe, at redshifts z > 7, have been found in large numbers with extremely deep restframe-UV imaging1. But it has proved difficult for existing spectrographs to characterise them in the UV2,3,4. The detailed properties of these galaxies could be measured from dust and cool gas emission at far-infrared wavelengths if the galaxies have become sufficiently enriched in dust and metals. So far, however, the most distant UV-selected galaxy detected in dust emission is only at z = 3.25, and recent results have cast doubt on whether dust and molecules can be found in typical galaxies at this early epoch6,7,8. Here we report thermal dust emission from an archetypal early universe star-forming galaxy, A1689-zD1. We detect its stellar continuum in spectroscopy and determine its redshift to be z = 7.5±0.2 from a spectroscopic detection of the Lyα break. A1689-zD1 is representative of the star-forming population during reionisation9, with a total star- –1 formation rate of about 12 M yr . The galaxy is highly evolved: it has a large stellar mass, and is heavily enriched in dust, with a dust-to-gas ratio close to that of the Milky Way. Dusty, evolved galaxies are thus present among the fainter star-forming population at z > 7, in spite of the very short time since they first appeared. -
Two Stellar Components in the Halo of the Milky Way
1 Two stellar components in the halo of the Milky Way Daniela Carollo1,2,3,5, Timothy C. Beers2,3, Young Sun Lee2,3, Masashi Chiba4, John E. Norris5 , Ronald Wilhelm6, Thirupathi Sivarani2,3, Brian Marsteller2,3, Jeffrey A. Munn7, Coryn A. L. Bailer-Jones8, Paola Re Fiorentin8,9, & Donald G. York10,11 1INAF - Osservatorio Astronomico di Torino, 10025 Pino Torinese, Italy, 2Department of Physics & Astronomy, Center for the Study of Cosmic Evolution, 3Joint Institute for Nuclear Astrophysics, Michigan State University, E. Lansing, MI 48824, USA, 4Astronomical Institute, Tohoku University, Sendai 980-8578, Japan, 5Research School of Astronomy & Astrophysics, The Australian National University, Mount Stromlo Observatory, Cotter Road, Weston Australian Capital Territory 2611, Australia, 6Department of Physics, Texas Tech University, Lubbock, TX 79409, USA, 7US Naval Observatory, P.O. Box 1149, Flagstaff, AZ 86002, USA, 8Max-Planck-Institute für Astronomy, Königstuhl 17, D-69117, Heidelberg, Germany, 9Department of Physics, University of Ljubljana, Jadronska 19, 1000, Ljubljana, Slovenia, 10Department of Astronomy and Astrophysics, Center, 11The Enrico Fermi Institute, University of Chicago, Chicago, IL, 60637, USA The halo of the Milky Way provides unique elemental abundance and kinematic information on the first objects to form in the Universe, which can be used to tightly constrain models of galaxy formation and evolution. Although the halo was once considered a single component, evidence for is dichotomy has slowly emerged in recent years from inspection of small samples of halo objects. Here we show that the halo is indeed clearly divisible into two broadly overlapping structural components -- an inner and an outer halo – that exhibit different spatial density profiles, stellar orbits and stellar metallicities (abundances of elements heavier than helium). -
The Large Scale Universe As a Quasi Quantum White Hole
International Astronomy and Astrophysics Research Journal 3(1): 22-42, 2021; Article no.IAARJ.66092 The Large Scale Universe as a Quasi Quantum White Hole U. V. S. Seshavatharam1*, Eugene Terry Tatum2 and S. Lakshminarayana3 1Honorary Faculty, I-SERVE, Survey no-42, Hitech city, Hyderabad-84,Telangana, India. 2760 Campbell Ln. Ste 106 #161, Bowling Green, KY, USA. 3Department of Nuclear Physics, Andhra University, Visakhapatnam-03, AP, India. Authors’ contributions This work was carried out in collaboration among all authors. Author UVSS designed the study, performed the statistical analysis, wrote the protocol, and wrote the first draft of the manuscript. Authors ETT and SL managed the analyses of the study. All authors read and approved the final manuscript. Article Information Editor(s): (1) Dr. David Garrison, University of Houston-Clear Lake, USA. (2) Professor. Hadia Hassan Selim, National Research Institute of Astronomy and Geophysics, Egypt. Reviewers: (1) Abhishek Kumar Singh, Magadh University, India. (2) Mohsen Lutephy, Azad Islamic university (IAU), Iran. (3) Sie Long Kek, Universiti Tun Hussein Onn Malaysia, Malaysia. (4) N.V.Krishna Prasad, GITAM University, India. (5) Maryam Roushan, University of Mazandaran, Iran. Complete Peer review History: http://www.sdiarticle4.com/review-history/66092 Received 17 January 2021 Original Research Article Accepted 23 March 2021 Published 01 April 2021 ABSTRACT We emphasize the point that, standard model of cosmology is basically a model of classical general relativity and it seems inevitable to have a revision with reference to quantum model of cosmology. Utmost important point to be noted is that, ‘Spin’ is a basic property of quantum mechanics and ‘rotation’ is a very common experience. -
An Outline of Stellar Astrophysics with Problems and Solutions
An Outline of Stellar Astrophysics with Problems and Solutions Using Maple R and Mathematica R Robert Roseberry 2016 1 Contents 1 Introduction 5 2 Electromagnetic Radiation 7 2.1 Specific intensity, luminosity and flux density ............7 Problem 1: luminous flux (**) . .8 Problem 2: galaxy fluxes (*) . .8 Problem 3: radiative pressure (**) . .9 2.2 Magnitude ...................................9 Problem 4: magnitude (**) . 10 2.3 Colour ..................................... 11 Problem 5: Planck{Stefan-Boltzmann{Wien{colour (***) . 13 Problem 6: Planck graph (**) . 13 Problem 7: radio and visual luminosity and brightness (***) . 14 Problem 8: Sirius (*) . 15 2.4 Emission Mechanisms: Continuum Emission ............. 15 Problem 9: Orion (***) . 17 Problem 10: synchrotron (***) . 18 Problem 11: Crab (**) . 18 2.5 Emission Mechanisms: Line Emission ................. 19 Problem 12: line spectrum (*) . 20 2.6 Interference: Line Broadening, Scattering, and Zeeman splitting 21 Problem 13: natural broadening (**) . 21 Problem 14: Doppler broadening (*) . 22 Problem 15: Thomson Cross Section (**) . 23 Problem 16: Inverse Compton scattering (***) . 24 Problem 17: normal Zeeman splitting (**) . 25 3 Measuring Distance 26 3.1 Parallax .................................... 27 Problem 18: parallax (*) . 27 3.2 Doppler shifting ............................... 27 Problem 19: supernova distance (***) . 28 3.3 Spectroscopic parallax and Main Sequence fitting .......... 28 Problem 20: Main Sequence fitting (**) . 29 3.4 Standard candles ............................... 30 Video: supernova light curve . 30 Problem 21: Cepheid distance (*) . 30 3.5 Tully-Fisher relation ............................ 31 3.6 Lyman-break galaxies and the Hubble flow .............. 33 4 Transparent Gas: Interstellar Gas Clouds and the Atmospheres and Photospheres of Stars 35 2 4.1 Transfer equation and optical depth .................. 36 Problem 22: optical depth (**) . 37 4.2 Plane-parallel atmosphere, Eddington's approximation, and limb darkening .................................. -
Virgo the Virgin
Virgo the Virgin Virgo is one of the constellations of the zodiac, the group tion Virgo itself. There is also the connection here with of 12 constellations that lies on the ecliptic plane defined “The Scales of Justice” and the sign Libra which lies next by the planets orbital orientation around the Sun. Virgo is to Virgo in the Zodiac. The study of astronomy had a one of the original 48 constellations charted by Ptolemy. practical “time keeping” aspect in the cultures of ancient It is the largest constellation of the Zodiac and the sec- history and as the stars of Virgo appeared before sunrise ond - largest constellation after Hydra. Virgo is bordered by late in the northern summer, many cultures linked this the constellations of Bootes, Coma Berenices, Leo, Crater, asterism with crops, harvest and fecundity. Corvus, Hydra, Libra and Serpens Caput. The constella- tion of Virgo is highly populated with galaxies and there Virgo is usually depicted with angel - like wings, with an are several galaxy clusters located within its boundaries, ear of wheat in her left hand, marked by the bright star each of which is home to hundreds or even thousands of Spica, which is Latin for “ear of grain”, and a tall blade of galaxies. The accepted abbreviation when enumerating grass, or a palm frond, in her right hand. Spica will be objects within the constellation is Vir, the genitive form is important for us in navigating Virgo in the modern night Virginis and meteor showers that appear to originate from sky. Spica was most likely the star that helped the Greek Virgo are called Virginids. -
And Ecclesiastical Cosmology
GSJ: VOLUME 6, ISSUE 3, MARCH 2018 101 GSJ: Volume 6, Issue 3, March 2018, Online: ISSN 2320-9186 www.globalscientificjournal.com DEMOLITION HUBBLE'S LAW, BIG BANG THE BASIS OF "MODERN" AND ECCLESIASTICAL COSMOLOGY Author: Weitter Duckss (Slavko Sedic) Zadar Croatia Pусскй Croatian „If two objects are represented by ball bearings and space-time by the stretching of a rubber sheet, the Doppler effect is caused by the rolling of ball bearings over the rubber sheet in order to achieve a particular motion. A cosmological red shift occurs when ball bearings get stuck on the sheet, which is stretched.“ Wikipedia OK, let's check that on our local group of galaxies (the table from my article „Where did the blue spectral shift inside the universe come from?“) galaxies, local groups Redshift km/s Blueshift km/s Sextans B (4.44 ± 0.23 Mly) 300 ± 0 Sextans A 324 ± 2 NGC 3109 403 ± 1 Tucana Dwarf 130 ± ? Leo I 285 ± 2 NGC 6822 -57 ± 2 Andromeda Galaxy -301 ± 1 Leo II (about 690,000 ly) 79 ± 1 Phoenix Dwarf 60 ± 30 SagDIG -79 ± 1 Aquarius Dwarf -141 ± 2 Wolf–Lundmark–Melotte -122 ± 2 Pisces Dwarf -287 ± 0 Antlia Dwarf 362 ± 0 Leo A 0.000067 (z) Pegasus Dwarf Spheroidal -354 ± 3 IC 10 -348 ± 1 NGC 185 -202 ± 3 Canes Venatici I ~ 31 GSJ© 2018 www.globalscientificjournal.com GSJ: VOLUME 6, ISSUE 3, MARCH 2018 102 Andromeda III -351 ± 9 Andromeda II -188 ± 3 Triangulum Galaxy -179 ± 3 Messier 110 -241 ± 3 NGC 147 (2.53 ± 0.11 Mly) -193 ± 3 Small Magellanic Cloud 0.000527 Large Magellanic Cloud - - M32 -200 ± 6 NGC 205 -241 ± 3 IC 1613 -234 ± 1 Carina Dwarf 230 ± 60 Sextans Dwarf 224 ± 2 Ursa Minor Dwarf (200 ± 30 kly) -247 ± 1 Draco Dwarf -292 ± 21 Cassiopeia Dwarf -307 ± 2 Ursa Major II Dwarf - 116 Leo IV 130 Leo V ( 585 kly) 173 Leo T -60 Bootes II -120 Pegasus Dwarf -183 ± 0 Sculptor Dwarf 110 ± 1 Etc. -
Galactic Metal-Poor Halo E NCYCLOPEDIA of a STRONOMY and a STROPHYSICS
Galactic Metal-Poor Halo E NCYCLOPEDIA OF A STRONOMY AND A STROPHYSICS Galactic Metal-Poor Halo Most of the gas, stars and clusters in our Milky Way Galaxy are distributed in its rotating, metal-rich, gas-rich and flattened disk and in the more slowly rotating, metal- rich and gas-poor bulge. The Galaxy’s halo is roughly spheroidal in shape, and extends, with decreasing density, out to distances comparable with those of the Magellanic Clouds and the dwarf spheroidal galaxies that have been collected around the Galaxy. Aside from its roughly spheroidal distribution, the most salient general properties of the halo are its low metallicity relative to the bulk of the Galaxy’s stars, its lack of a gaseous counterpart, unlike the Galactic disk, and its great age. The kinematics of the stellar halo is closely coupled to the spheroidal distribution. Solar neighborhood disk stars move at a speed of about 220 km s−1 toward a point in the plane ◦ and 90 from the Galactic center. Stars belonging to the spheroidal halo do not share such ordered motion, and thus appear to have ‘high velocities’ relative to the Sun. Their orbital energies are often comparable with those of the disk stars but they are directed differently, often on orbits that have a smaller component of rotation or angular Figure 1. The distribution of [Fe/H] values for globular clusters. momentum. Following the original description by Baade in 1944, the disk stars are often called POPULATION I while still used to measure R . The recognizability of globular the metal-poor halo stars belong to POPULATION II. -
Compact Stars
Compact Stars Lecture 12 Summary of the previous lecture I talked about neutron stars, their internel structure, the types of equation of state, and resulting maximum mass (Tolman-Openheimer-Volkoff limit) The neutron star EoS can be constrained if we know mass and/or radius of a star from observations Neutron stars exist in isolation, or are in binaries with MS stars, compact stars, including other NS. These binaries are final product of common evolution of the binary system, or may be a result of capture. Binary NS-NS merger leads to emission of gravitational waves, and also to the short gamma ray burst. The follow up may be observed as a ’kilonova’ due to radioactive decay of high-mass neutron-rich isotopes ejected from merger GRBs and cosmology In 1995, the 'Diamond Jubilee' debate was organized to present the issue of distance scale to GRBs It was a remainder of the famous debate in 1920 between Curtis and Shapley. Curtis argued that the Universe is composed of many galaxies like our own, identified as ``spiral nebulae". Shapley argued that these ``spiral nebulae" were just nearby gas clouds, and that the Universe was composed of only one big Galaxy. Now, Donald Lamb argued that the GRB sources were in the galactic halo while Bodhan Paczyński argued that they were at cosmological distances. B. Paczyński, M. Rees and D. Lamb GRBs and cosmology The GRBs should be easily detectable out to z=20 (Lamb & Reichart 2000). GRB 090429: z=9.4 The IR afterglows of long GRBs can be used as probes of very high z Universe, due to combined effects of cosmological dilation and decrease of intensity with time. -
Dwarf Galaxies 1 Planck “Merger Tree” Hierarchical Structure Formation
04.04.2019 Grebel: Dwarf Galaxies 1 Planck “Merger Tree” Hierarchical Structure Formation q Larger structures form q through successive Illustris q mergers of smaller simulation q structures. q If baryons are Time q involved: Observable q signatures of past merger q events may be retained. ➙ Dwarf galaxies as building blocks of massive galaxies. Potentially traceable; esp. in galactic halos. Fundamental scenario: q Surviving dwarfs: Fossils of galaxy formation q and evolution. Large structures form through numerous mergers of smaller ones. 04.04.2019 Grebel: Dwarf Galaxies 2 Satellite Disruption and Accretion Satellite disruption: q may lead to tidal q stripping (up to 90% q of the satellite’s original q stellar mass may be lost, q but remnant may survive), or q to complete disruption and q ultimately satellite accretion. Harding q More massive satellites experience Stellar tidal streams r r q higher dynamical friction dV M ρ V from different dwarf ∝ − r 3 galaxy accretion q and sink more rapidly. dt V events lead to ➙ Due to the mass-metallicity relation, expect a highly sub- q more metal-rich stars to end up at smaller radii. structured halo. 04.04.2019 € Grebel: Dwarf Galaxies Johnston 3 De Lucia & Helmi 2008; Cooper et al. 2010 accreted stars (ex situ) in-situ stars Stellar Halo Origins q Stellar halos composed in part of q accreted stars and in part of stars q formed in situ. Rodriguez- q Halos grow from “from inside out”. Gomez et al. 2016 q Wide variety of satellite accretion histories from smooth growth to discrete events. -
Monthly Newsletter of the Durban Centre - March 2018
Page 1 Monthly Newsletter of the Durban Centre - March 2018 Page 2 Table of Contents Chairman’s Chatter …...…………………….……….………..….…… 3 Andrew Gray …………………………………………...………………. 5 The Hyades Star Cluster …...………………………….…….……….. 6 At the Eye Piece …………………………………………….….…….... 9 The Cover Image - Antennae Nebula …….……………………….. 11 Galaxy - Part 2 ….………………………………..………………….... 13 Self-Taught Astronomer …………………………………..………… 21 The Month Ahead …..…………………...….…….……………..…… 24 Minutes of the Previous Meeting …………………………….……. 25 Public Viewing Roster …………………………….……….…..……. 26 Pre-loved Telescope Equipment …………………………...……… 28 ASSA Symposium 2018 ………………………...……….…......…… 29 Member Submissions Disclaimer: The views expressed in ‘nDaba are solely those of the writer and are not necessarily the views of the Durban Centre, nor the Editor. All images and content is the work of the respective copyright owner Page 3 Chairman’s Chatter By Mike Hadlow Dear Members, The third month of the year is upon us and already the viewing conditions have been more favourable over the last few nights. Let’s hope it continues and we have clear skies and good viewing for the next five or six months. Our February meeting was well attended, with our main speaker being Dr Matt Hilton from the Astrophysics and Cosmology Research Unit at UKZN who gave us an excellent presentation on gravity waves. We really have to be thankful to Dr Hilton from ACRU UKZN for giving us his time to give us presentations and hope that we can maintain our relationship with ACRU and that we can draw other speakers from his colleagues and other research students! Thanks must also go to Debbie Abel and Piet Strauss for their monthly presentations on NASA and the sky for the following month, respectively. -
Stacking Emission Lines from Distant Galaxies
DF A study of galaxy evolution: stacking emission lines from distant galaxies JEAN-BAPTISTE JOLLY Department of Space Earth and Environment CHALMERS UNIVERSITY OF TECHNOLOGY Gothenburg, Sweden 2019 Thesis for the degree of Licentiate of Engineering A study of galaxy evolution: stacking emission lines from distant galaxies Jean-Baptiste Jolly DF Department of Space Earth and Environment Division of Astronomy and Plasma Physics Chalmers University of Technology Gothenburg, Sweden 2019 A study of galaxy evolution: stacking emission lines from distant galaxies Jean-Baptiste Jolly © Jean-Baptiste Jolly, 2019. Division of Astronomy and Plasma Physics Department of Space Earth and Environment Chalmers University of Technology SE-412 96 Gothenburg Telephone +46 31 772 1000 Contact information: Jean-Baptiste Jolly Onsala Space Observatory Chalmers University of Technology SE–439 92 Onsala, Sweden Phone: +46 (0)31 772 55 44 Email: [email protected] Cover image: This image from the NASA/ESA Hubble Space Telescope shows the galaxy cluster MACSJ0717.5+3745. This is one of six being studied by the Hub- ble Frontier Fields programme, which together have produced the deepest images of gravitational lensing ever made. Due to the huge mass of the cluster it is bending the light of background objects, acting as a magnifying lens. It is one of the most massive galaxy clusters known, and it is also the largest known gravitational lens. Of all of the galaxy clusters known and measured, MACS J0717 lenses the largest area of the sky. Credit: NASA, ESA and the HST Frontier Fields team (STScI) Printed by Chalmers Reproservice Gothenburg, Sweden 2019 A study of galaxy evolution: stacking emission lines from distant galaxies Jean-Baptiste Jolly Department of Space Earth and Environment Chalmers University of Technology Abstract To draw up a thorough description of galaxy evolution exhaustive observations are needed, of distant but mainly of faint galaxies. -
The Evolution of Galaxy Morphology
The Morphological Evolution of Galaxies Roberto G. Abraham Sidney van den Bergh Dept. of Astronomy & Dominion Astrophysical Observatory Astrophysics Herzberg Institute of Astrophysics University of Toronto National Research Council of Canada 60 St. George Street Victoria, British Columbia Toronto, Ontario M5S 3H8, V9E 2E7, Canada Canada ABSTRACT Many galaxies appear to have taken on their familiar appearance relatively recently. In the distant Universe, galaxy morphology started to deviate significantly (and systematically) from that of nearby galaxies at redshifts, z, as low as z = 0.3. This corresponds to a time ~3.5 Gyr in the past, which is only ~25% of the present age of the Universe. Beyond z = 0.5 (5 Gyr in the past) spiral arms are less well-developed and more chaotic, and barred spiral galaxies may become rarer. By z = 1, around 30% of the galaxy population is sufficiently peculiar that classification on Hubble’s traditional “tuning fork” system is meaningless. On the other hand, some characteristics of galaxies do not seem to have changed much over time. The co-moving space density of luminous disk galaxies has not changed significantly since z = 1, indicating that while the general appearance of these objects has continuously changed with cosmic epoch, their overall numbers have been conserved. Attempts to explain these results with hierarchical models for the formation of galaxies have met with mixed success. denoted Sa, Sb, and Sc (SBa, SBb, SBc in of Hubble’s classification system are Introduction the case of barred spirals). A final “catch- based on the idea that most matter in the all” category for irregular galaxies is also Universe is not in stellar or gaseous form, Nearby galaxies are usually classified on included.