Batf Defines a Differentiation Checkpoint Limiting Hematopoietic Stem Cell Self Renewal in Response to DNA Damage
Total Page:16
File Type:pdf, Size:1020Kb
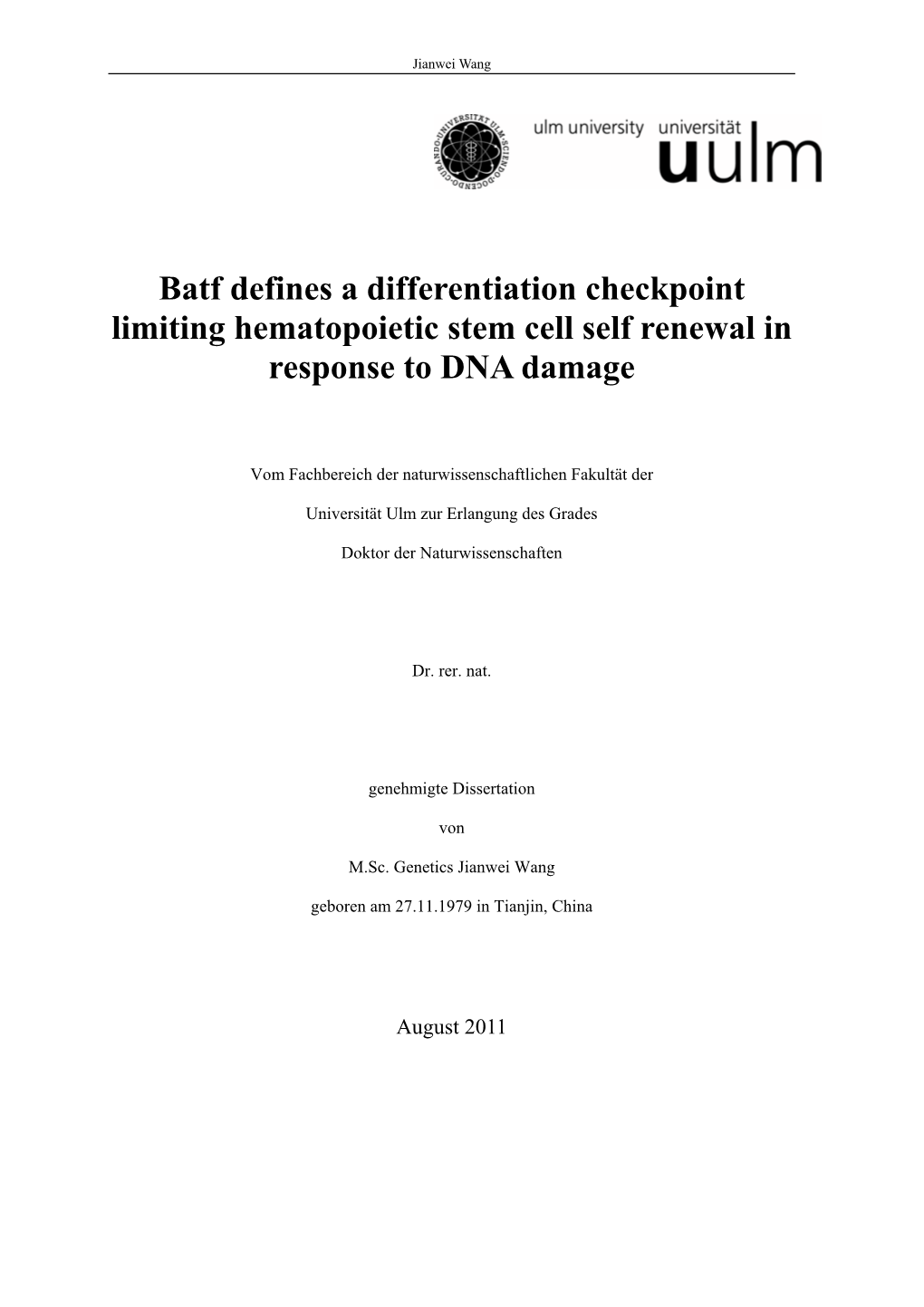
Load more
Recommended publications
-
A Computational Approach for Defining a Signature of Β-Cell Golgi Stress in Diabetes Mellitus
Page 1 of 781 Diabetes A Computational Approach for Defining a Signature of β-Cell Golgi Stress in Diabetes Mellitus Robert N. Bone1,6,7, Olufunmilola Oyebamiji2, Sayali Talware2, Sharmila Selvaraj2, Preethi Krishnan3,6, Farooq Syed1,6,7, Huanmei Wu2, Carmella Evans-Molina 1,3,4,5,6,7,8* Departments of 1Pediatrics, 3Medicine, 4Anatomy, Cell Biology & Physiology, 5Biochemistry & Molecular Biology, the 6Center for Diabetes & Metabolic Diseases, and the 7Herman B. Wells Center for Pediatric Research, Indiana University School of Medicine, Indianapolis, IN 46202; 2Department of BioHealth Informatics, Indiana University-Purdue University Indianapolis, Indianapolis, IN, 46202; 8Roudebush VA Medical Center, Indianapolis, IN 46202. *Corresponding Author(s): Carmella Evans-Molina, MD, PhD ([email protected]) Indiana University School of Medicine, 635 Barnhill Drive, MS 2031A, Indianapolis, IN 46202, Telephone: (317) 274-4145, Fax (317) 274-4107 Running Title: Golgi Stress Response in Diabetes Word Count: 4358 Number of Figures: 6 Keywords: Golgi apparatus stress, Islets, β cell, Type 1 diabetes, Type 2 diabetes 1 Diabetes Publish Ahead of Print, published online August 20, 2020 Diabetes Page 2 of 781 ABSTRACT The Golgi apparatus (GA) is an important site of insulin processing and granule maturation, but whether GA organelle dysfunction and GA stress are present in the diabetic β-cell has not been tested. We utilized an informatics-based approach to develop a transcriptional signature of β-cell GA stress using existing RNA sequencing and microarray datasets generated using human islets from donors with diabetes and islets where type 1(T1D) and type 2 diabetes (T2D) had been modeled ex vivo. To narrow our results to GA-specific genes, we applied a filter set of 1,030 genes accepted as GA associated. -
Supplemental Materials ZNF281 Enhances Cardiac Reprogramming
Supplemental Materials ZNF281 enhances cardiac reprogramming by modulating cardiac and inflammatory gene expression Huanyu Zhou, Maria Gabriela Morales, Hisayuki Hashimoto, Matthew E. Dickson, Kunhua Song, Wenduo Ye, Min S. Kim, Hanspeter Niederstrasser, Zhaoning Wang, Beibei Chen, Bruce A. Posner, Rhonda Bassel-Duby and Eric N. Olson Supplemental Table 1; related to Figure 1. Supplemental Table 2; related to Figure 1. Supplemental Table 3; related to the “quantitative mRNA measurement” in Materials and Methods section. Supplemental Table 4; related to the “ChIP-seq, gene ontology and pathway analysis” and “RNA-seq” and gene ontology analysis” in Materials and Methods section. Supplemental Figure S1; related to Figure 1. Supplemental Figure S2; related to Figure 2. Supplemental Figure S3; related to Figure 3. Supplemental Figure S4; related to Figure 4. Supplemental Figure S5; related to Figure 6. Supplemental Table S1. Genes included in human retroviral ORF cDNA library. Gene Gene Gene Gene Gene Gene Gene Gene Symbol Symbol Symbol Symbol Symbol Symbol Symbol Symbol AATF BMP8A CEBPE CTNNB1 ESR2 GDF3 HOXA5 IL17D ADIPOQ BRPF1 CEBPG CUX1 ESRRA GDF6 HOXA6 IL17F ADNP BRPF3 CERS1 CX3CL1 ETS1 GIN1 HOXA7 IL18 AEBP1 BUD31 CERS2 CXCL10 ETS2 GLIS3 HOXB1 IL19 AFF4 C17ORF77 CERS4 CXCL11 ETV3 GMEB1 HOXB13 IL1A AHR C1QTNF4 CFL2 CXCL12 ETV7 GPBP1 HOXB5 IL1B AIMP1 C21ORF66 CHIA CXCL13 FAM3B GPER HOXB6 IL1F3 ALS2CR8 CBFA2T2 CIR1 CXCL14 FAM3D GPI HOXB7 IL1F5 ALX1 CBFA2T3 CITED1 CXCL16 FASLG GREM1 HOXB9 IL1F6 ARGFX CBFB CITED2 CXCL3 FBLN1 GREM2 HOXC4 IL1F7 -
Transcriptional Repression of IFN Regulatory Factor 7 by MYC Is Critical for Type I IFN Production in Human Plasmacytoid Dendrit
Transcriptional Repression of IFN Regulatory Factor 7 by MYC Is Critical for Type I IFN Production in Human Plasmacytoid Dendritic Cells This information is current as of September 28, 2021. Tae Whan Kim, Seunghee Hong, Yin Lin, Elise Murat, HyeMee Joo, Taeil Kim, Virginia Pascual and Yong-Jun Liu J Immunol 2016; 197:3348-3359; Prepublished online 14 September 2016; doi: 10.4049/jimmunol.1502385 Downloaded from http://www.jimmunol.org/content/197/8/3348 Supplementary http://www.jimmunol.org/content/suppl/2016/09/14/jimmunol.150238 http://www.jimmunol.org/ Material 5.DCSupplemental References This article cites 74 articles, 25 of which you can access for free at: http://www.jimmunol.org/content/197/8/3348.full#ref-list-1 Why The JI? Submit online. • Rapid Reviews! 30 days* from submission to initial decision by guest on September 28, 2021 • No Triage! Every submission reviewed by practicing scientists • Fast Publication! 4 weeks from acceptance to publication *average Subscription Information about subscribing to The Journal of Immunology is online at: http://jimmunol.org/subscription Permissions Submit copyright permission requests at: http://www.aai.org/About/Publications/JI/copyright.html Email Alerts Receive free email-alerts when new articles cite this article. Sign up at: http://jimmunol.org/alerts The Journal of Immunology is published twice each month by The American Association of Immunologists, Inc., 1451 Rockville Pike, Suite 650, Rockville, MD 20852 Copyright © 2016 by The American Association of Immunologists, Inc. All rights reserved. Print ISSN: 0022-1767 Online ISSN: 1550-6606. The Journal of Immunology Transcriptional Repression of IFN Regulatory Factor 7 by MYC Is Critical for Type I IFN Production in Human Plasmacytoid Dendritic Cells Tae Whan Kim,*,1 Seunghee Hong,*,1 Yin Lin,* Elise Murat,* HyeMee Joo,* Taeil Kim,†,2 Virginia Pascual,* and Yong-Jun Liu*,† Type I IFNs are crucial mediators of human innate and adaptive immunity and are massively produced from plasmacytoid dendritic cells (pDCs). -
Interferon-Α Therapy in Bcr-Abl-Negative Myeloproliferative Neoplasms
Leukemia (2008) 22, 1990–1998 & 2008 Macmillan Publishers Limited All rights reserved 0887-6924/08 $32.00 www.nature.com/leu SPOTLIGHT REVIEW Interferon-a therapy in bcr-abl-negative myeloproliferative neoplasms J-J Kiladjian1,2,3, C Chomienne4 and P Fenaux1 1Assistance PubliqueFHoˆpitaux de Paris, Hoˆpital Avicenne, Service d’He´matologie Clinique, Bobigny, France; 2Assistance PubliqueFHoˆpitaux de Paris, Hoˆpital Saint-Louis, Centre d’Investigation Clinique, Paris, France; 3Universite´ Paris 7, Denis Diderot, Paris, France and 4Assistance PubliqueFHoˆpitaux de Paris, Hoˆpital Saint-Louis, Unite´ de Biologie Cellulaire, Paris, France Interferon (IFN) was the first cytokine discovered 50 years ago, however, did not allow complete purity, and although leukocyte with a wide range of biological properties, including immuno- IFN consisted predominantly of IFN-a, small amounts of other modulatory, proapoptotic and antiangiogenic activities, that rapidly raised interest in its therapeutic use in malignancies. IFN species were also present. Later on, the cloning of IFN-receptor characterization was also pivotal in the discovery recombinant human IFN-a and -b in 1980 allowed the SPOTLIGHT of the JAK/STAT signaling pathway. Among the large IFN production of large amounts of IFN for characterization, family, mainly one of the type I IFN, IFN-a2, is used in therapy. biological activity studies and clinical trials.5–7 It is only 20 Many clinical trials have shown remarkable efficacy of IFN-a in years after their discovery that two IFN species, IFN-a and -b, bcr-abl-negative myeloproliferative neoplasms (MPNs), espe- could be purified satisfactorily using high-performance liquid cially polycythemia vera (PV), and essential thrombocythemia 8 (ET). -
Supplemental Table S1 (A): Microarray Datasets Characteristics
Supplemental table S1 (A): Microarray datasets characteristics Title Summary Samples Literature ref. GEO ref. Acquisition of granule Gene expression profiling of 27 (1) GSE 11859 neuron precursor identity cerebellar tumors generated and Hedgehog‐induced from various early and late medulloblastoma in mice. stage CNS progenitor cells Medulloblastomas derived Study of mouse 5 (2) GSE 7212 from Cxcr6 mutant mice medulloblastoma in response respond to treatment with to inhibitor of Smoothened a Smoothened inhibitor Expression profiles of Identification of distinct classes 10 (3) GSE 9299 mouse medulloblastoma of up‐regulated or down‐ 339 & 340 regulated genes during Hh dependent tumorigenesis Genetic alterations in Identification of differently 10 (4) GSE 6463 mouse medulloblastomas expressed genes among CGNPs 339 & and generation of tumors and CGNPs transfected with 340 from cerebellar granule retroviruses that express nmyc neuron precursors or cyclin‐d1 Patched heterozygous Analysis of granule cell 14 (5) GSE 2426 model of medulloblastoma precursors, pre‐neoplastic cells, GDS1110 and tumor cells 1. Schuller U, Heine VM, Mao J, Kho AT, Dillon AK, Han YG, et al. Acquisition of granule neuron precursor identity is a critical determinant of progenitor cell competence to form Shh‐induced medulloblastoma. Cancer Cell 2008;14:123‐134. 2. Sasai K, Romer JT, Kimura H, Eberhart DE, Rice DS, Curran T. Medulloblastomas derived from Cxcr6 mutant mice respond to treatment with a smoothened inhibitor. Cancer Res 2007;67:3871‐3877. 3. Mao J, Ligon KL, Rakhlin EY, Thayer SP, Bronson RT, Rowitch D, et al. A novel somatic mouse model to survey tumorigenic potential applied to the Hedgehog pathway. Cancer Res 2006;66:10171‐10178. -
Type I Interferons in Anticancer Immunity
REVIEWS Type I interferons in anticancer immunity Laurence Zitvogel1–4*, Lorenzo Galluzzi1,5–8*, Oliver Kepp5–9, Mark J. Smyth10,11 and Guido Kroemer5–9,12 Abstract | Type I interferons (IFNs) are known for their key role in antiviral immune responses. In this Review, we discuss accumulating evidence indicating that type I IFNs produced by malignant cells or tumour-infiltrating dendritic cells also control the autocrine or paracrine circuits that underlie cancer immunosurveillance. Many conventional chemotherapeutics, targeted anticancer agents, immunological adjuvants and oncolytic 1Gustave Roussy Cancer Campus, F-94800 Villejuif, viruses are only fully efficient in the presence of intact type I IFN signalling. Moreover, the France. intratumoural expression levels of type I IFNs or of IFN-stimulated genes correlate with 2INSERM, U1015, F-94800 Villejuif, France. favourable disease outcome in several cohorts of patients with cancer. Finally, new 3Université Paris Sud/Paris XI, anticancer immunotherapies are being developed that are based on recombinant type I IFNs, Faculté de Médecine, F-94270 Le Kremlin Bicêtre, France. type I IFN-encoding vectors and type I IFN-expressing cells. 4Center of Clinical Investigations in Biotherapies of Cancer (CICBT) 507, F-94800 Villejuif, France. Type I interferons (IFNs) were first discovered more than Type I IFNs in cancer immunosurveillance 5Equipe 11 labellisée par la half a century ago as the factors underlying viral inter Type I IFNs are known to mediate antineoplastic effects Ligue Nationale contre le ference — that is, the ability of a primary viral infection against several malignancies, which is a clinically rel Cancer, Centre de Recherche 1 des Cordeliers, F-75006 Paris, to render cells resistant to a second distinct virus . -
Heatmaps - the Gene Expression Edition
Heatmaps - the gene expression edition Jeff Oliver 20 July, 2020 An application of heatmap visualization to investigate differential gene expression. Learning objectives 1. Manipulate data into a ‘tidy’ format 2. Visualize data in a heatmap 3. Become familiar with ggplot syntax for customizing plots Heatmaps for differential gene expression Heatmaps are a great way of displaying three-dimensional data in only two dimensions. But how can we easily translate tabular data into a format for heatmap plotting? By taking advantage of “data munging” and graphics packages, heatmaps are relatively easy to produce in R. Getting started We are going to start by isolating different types of information by imposing structure in our file managment. That is, we are going to put our input data in one folder and any output such as plots or analytical results in a different folder. We can use the dir.create to create these two folders: dir.create("data") dir.create("output") For this lesson, we will use a subset of data on a study of gene expression in cells infected with the influenza virus (doi: 10.4049/jimmunol.1501557). The study infected human plasmacytoid dendritic cells with the influenza virus, and compared gene expression in those cells to gene expression in uninfected cells. Thegoal was to see how the flu virus affected the function of these immune system cells. The data for this lesson is available at: http://tinyurl.com/flu-expression-data or https://jcoliver.github.io/ learn-r/data/GSE68849-expression.csv. Download this comma separated file and put it in the data folder. -
Supplementary Table S4. FGA Co-Expressed Gene List in LUAD
Supplementary Table S4. FGA co-expressed gene list in LUAD tumors Symbol R Locus Description FGG 0.919 4q28 fibrinogen gamma chain FGL1 0.635 8p22 fibrinogen-like 1 SLC7A2 0.536 8p22 solute carrier family 7 (cationic amino acid transporter, y+ system), member 2 DUSP4 0.521 8p12-p11 dual specificity phosphatase 4 HAL 0.51 12q22-q24.1histidine ammonia-lyase PDE4D 0.499 5q12 phosphodiesterase 4D, cAMP-specific FURIN 0.497 15q26.1 furin (paired basic amino acid cleaving enzyme) CPS1 0.49 2q35 carbamoyl-phosphate synthase 1, mitochondrial TESC 0.478 12q24.22 tescalcin INHA 0.465 2q35 inhibin, alpha S100P 0.461 4p16 S100 calcium binding protein P VPS37A 0.447 8p22 vacuolar protein sorting 37 homolog A (S. cerevisiae) SLC16A14 0.447 2q36.3 solute carrier family 16, member 14 PPARGC1A 0.443 4p15.1 peroxisome proliferator-activated receptor gamma, coactivator 1 alpha SIK1 0.435 21q22.3 salt-inducible kinase 1 IRS2 0.434 13q34 insulin receptor substrate 2 RND1 0.433 12q12 Rho family GTPase 1 HGD 0.433 3q13.33 homogentisate 1,2-dioxygenase PTP4A1 0.432 6q12 protein tyrosine phosphatase type IVA, member 1 C8orf4 0.428 8p11.2 chromosome 8 open reading frame 4 DDC 0.427 7p12.2 dopa decarboxylase (aromatic L-amino acid decarboxylase) TACC2 0.427 10q26 transforming, acidic coiled-coil containing protein 2 MUC13 0.422 3q21.2 mucin 13, cell surface associated C5 0.412 9q33-q34 complement component 5 NR4A2 0.412 2q22-q23 nuclear receptor subfamily 4, group A, member 2 EYS 0.411 6q12 eyes shut homolog (Drosophila) GPX2 0.406 14q24.1 glutathione peroxidase -
FOXN3 Inhibits Cell Proliferation Though Regulating E2F1 in Prostate Cancer
Research Paper FOXN3 Inhibits Cell Proliferation though Regulating E2F1 in Prostate Cancer NING. ZHANG, HAIJUN. YANG* AND QINGQI. ZENG Department of Traditional Chinese Medicine, Jiangsu Health Vocational College, No. 69 Huangshanling Road, Nanjing, Jiangsu 211800, China Zhang et al.: FOXN3 Inhibits Cell Proliferation in Prostate Cancer In this study, it was demonstrated that forkhead box N3 was downregulated in prostate cancer tissues compared to the matched normal adjacent tissues. Prostate cancer patients with high forkhead box N3 expression more frequently had longer survival (P<0.05) than those with low forkhead box N3 expression. Forkhead box N3 inhibited prostate cancer cell proliferation in vitro and in vivo. In addition, forkhead box N3 repressed expression of E2F1, a reported potential oncogene at the messenger ribonucleic acid and protein levels. Taken together, these data delineate an unrecognized function of forkhead box N3 as a tumor suppressor in prostate cancer via repressing the expression of E2F1. Key words: Prostate cancer, FOXN3, E2F1, cell proliferation As a common urogenital system malignant tumor in could down-regulate E2F5 in human cells to control men, prostate cancer (PC) threatens men’s health and cell cycle or inhibit protein biosynthesis[6]. In addition, becomes an important cause of death in the elderly[1]. studies have shown that FOXN3 is significantly The incidence of PC has increased in most countries downregulated in several cancer tissues including oral and PC represents a major disease burden worldwide in laryngeal carcinoma hepatocellular carcinoma, the past decade[2,3]. Treatment options for PC depended squamous cell carcinoma and colon cancer[6,9,10]. -
Appendix 2. Significantly Differentially Regulated Genes in Term Compared with Second Trimester Amniotic Fluid Supernatant
Appendix 2. Significantly Differentially Regulated Genes in Term Compared With Second Trimester Amniotic Fluid Supernatant Fold Change in term vs second trimester Amniotic Affymetrix Duplicate Fluid Probe ID probes Symbol Entrez Gene Name 1019.9 217059_at D MUC7 mucin 7, secreted 424.5 211735_x_at D SFTPC surfactant protein C 416.2 206835_at STATH statherin 363.4 214387_x_at D SFTPC surfactant protein C 295.5 205982_x_at D SFTPC surfactant protein C 288.7 1553454_at RPTN repetin solute carrier family 34 (sodium 251.3 204124_at SLC34A2 phosphate), member 2 238.9 206786_at HTN3 histatin 3 161.5 220191_at GKN1 gastrokine 1 152.7 223678_s_at D SFTPA2 surfactant protein A2 130.9 207430_s_at D MSMB microseminoprotein, beta- 99.0 214199_at SFTPD surfactant protein D major histocompatibility complex, class II, 96.5 210982_s_at D HLA-DRA DR alpha 96.5 221133_s_at D CLDN18 claudin 18 94.4 238222_at GKN2 gastrokine 2 93.7 1557961_s_at D LOC100127983 uncharacterized LOC100127983 93.1 229584_at LRRK2 leucine-rich repeat kinase 2 HOXD cluster antisense RNA 1 (non- 88.6 242042_s_at D HOXD-AS1 protein coding) 86.0 205569_at LAMP3 lysosomal-associated membrane protein 3 85.4 232698_at BPIFB2 BPI fold containing family B, member 2 84.4 205979_at SCGB2A1 secretoglobin, family 2A, member 1 84.3 230469_at RTKN2 rhotekin 2 82.2 204130_at HSD11B2 hydroxysteroid (11-beta) dehydrogenase 2 81.9 222242_s_at KLK5 kallikrein-related peptidase 5 77.0 237281_at AKAP14 A kinase (PRKA) anchor protein 14 76.7 1553602_at MUCL1 mucin-like 1 76.3 216359_at D MUC7 mucin 7, -
Transcriptional and Post-Transcriptional Regulation of ATP-Binding Cassette Transporter Expression
Transcriptional and Post-transcriptional Regulation of ATP-binding Cassette Transporter Expression by Aparna Chhibber DISSERTATION Submitted in partial satisfaction of the requirements for the degree of DOCTOR OF PHILOSOPHY in Pharmaceutical Sciences and Pbarmacogenomies in the Copyright 2014 by Aparna Chhibber ii Acknowledgements First and foremost, I would like to thank my advisor, Dr. Deanna Kroetz. More than just a research advisor, Deanna has clearly made it a priority to guide her students to become better scientists, and I am grateful for the countless hours she has spent editing papers, developing presentations, discussing research, and so much more. I would not have made it this far without her support and guidance. My thesis committee has provided valuable advice through the years. Dr. Nadav Ahituv in particular has been a source of support from my first year in the graduate program as my academic advisor, qualifying exam committee chair, and finally thesis committee member. Dr. Kathy Giacomini graciously stepped in as a member of my thesis committee in my 3rd year, and Dr. Steven Brenner provided valuable input as thesis committee member in my 2nd year. My labmates over the past five years have been incredible colleagues and friends. Dr. Svetlana Markova first welcomed me into the lab and taught me numerous laboratory techniques, and has always been willing to act as a sounding board. Michael Martin has been my partner-in-crime in the lab from the beginning, and has made my days in lab fly by. Dr. Yingmei Lui has made the lab run smoothly, and has always been willing to jump in to help me at a moment’s notice. -
Supplementary Table 5. Clover Results Indicate the Number Of
Supplementary Table 5. Clover results indicate the number of chromosomes with transcription factor binding motifs statistically over‐ or under‐represented in HTE DHS within intergenic sequence (more than 2kb outside of any gene). Analysis was divided into three groups (all DHS, HTE‐selective DHS, and ubiquitous DHS). Motifs with more than one entry in the databases utilized were edited to retain only the first occurrence of the motif. All DHS x Intergenic TEselective DHS x Intergenic Ubiquitous DHS x Intergenic ID Name p < 0.01 p > 0.99 ID Name p < 0.01 p > 0.99 ID Name p < 0.01 p > 0.99 MA0002.2 RUNX1 23 0 MA0080.2 SPI1 23 0 MA0055.1 Myf 23 0 MA0003.1 TFAP2A 23 0 MA0089.1 NFE2L1::MafG 23 0 MA0068.1 Pax4 23 0 MA0039.2 Klf4 23 0 MA0098.1 ETS1 23 0 MA0080.2 SPI1 23 0 MA0055.1 Myf 23 0 MA0099.2 AP1 23 0 MA0098.1 ETS1 23 0 MA0056.1 MZF1_1‐4 23 0 MA0136.1 ELF5 23 0 MA0139.1 CTCF 23 0 MA0079.2 SP1 23 0 MA0145.1 Tcfcp2l1 23 0 V$ALX3_01 ALX‐3 23 0 MA0080.2 SPI1 23 0 MA0150.1 NFE2L2 23 0 V$ALX4_02 Alx‐4 23 0 myocyte enhancer MA0081.1 SPIB 23 0 MA0156.1 FEV 23 0 V$AMEF2_Q6 factor 23 0 MA0089.1 NFE2L1::MafG 23 0 V$AP1FJ_Q2 activator protein 1 23 0 V$AP1_01 AP‐1 23 0 MA0090.1 TEAD1 23 0 V$AP4_Q5 activator protein 4 23 0 V$AP2_Q6_01 AP‐2 23 0 MA0098.1 ETS1 23 0 V$AR_Q6 half‐site matrix 23 0 V$ARX_01 Arx 23 0 BTB and CNC homolog MA0099.2 AP1 23 0 V$BACH1_01 1 23 0 V$BARHL1_01 Barhl‐1 23 0 BTB and CNC homolog MA0136.1 ELF5 23 0 V$BACH2_01 2 23 0 V$BARHL2_01 Barhl2 23 0 MA0139.1 CTCF 23 0 V$CMAF_02 C‐MAF 23 0 V$BARX1_01 Barx1 23 0 MA0144.1 Stat3 23 0