Early Life Supraphysiological Levels of Oxygen Exposure Permanently
Total Page:16
File Type:pdf, Size:1020Kb
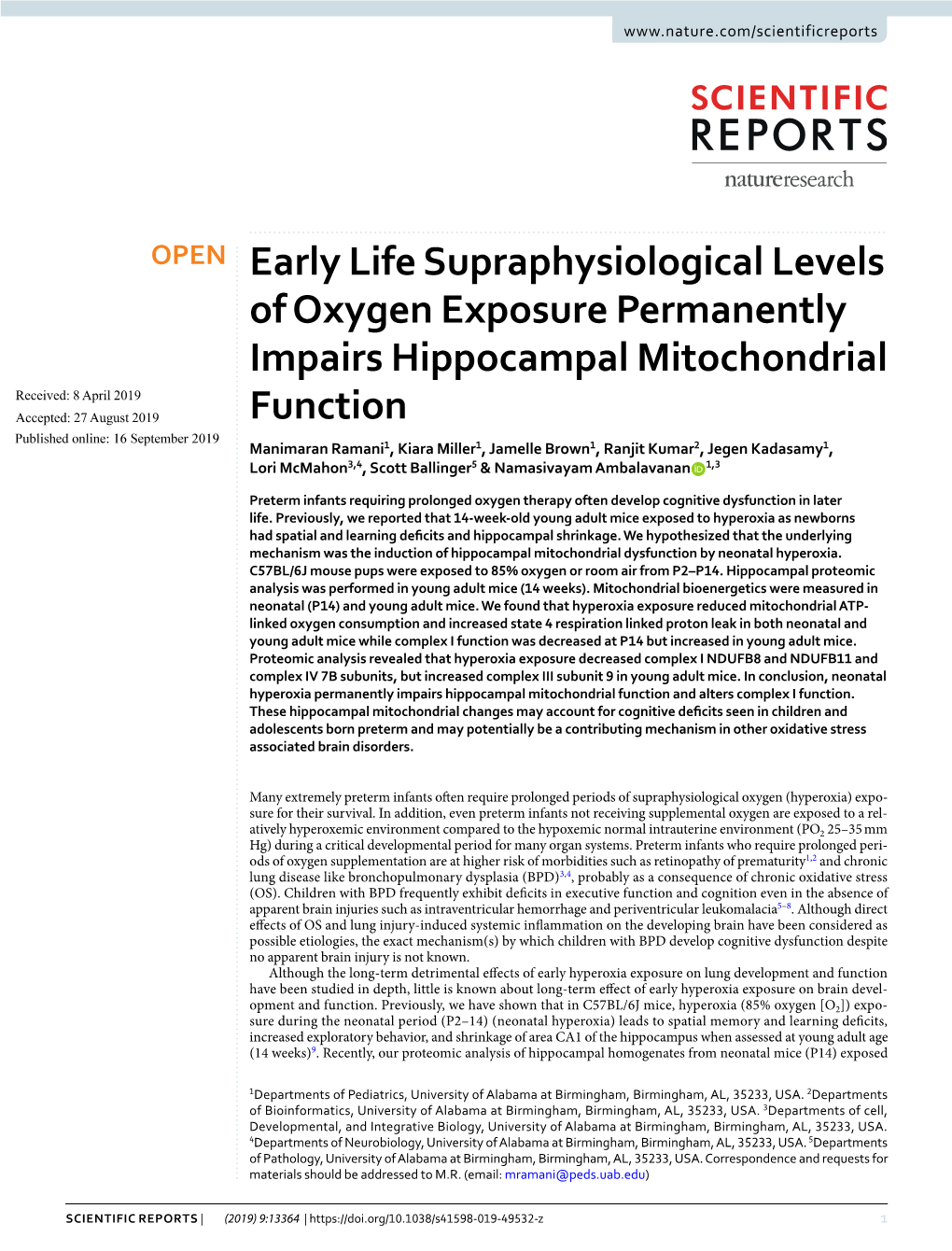
Load more
Recommended publications
-
(12) Patent Application Publication (10) Pub. No.: US 2012/0070450 A1 Ishikawa Et Al
US 20120070450A1 (19) United States (12) Patent Application Publication (10) Pub. No.: US 2012/0070450 A1 Ishikawa et al. (43) Pub. Date: Mar. 22, 2012 (54) LEUKEMA STEM CELLMARKERS Publication Classification (51) Int. Cl. A 6LX 39/395 (2006.01) (75) Inventors: Fumihiko Ishikawa, Kanagawa CI2O I/68 (2006.01) (JP): Osamu Ohara, Kanagawa GOIN 2L/64 (2006.01) (JP); Yoriko Saito, Kanagawa (JP); A6IP35/02 (2006.01) Hiroshi Kitamura, Kanagawa (JP); C40B 30/04 (2006.01) Atsushi Hijikata, Kanagawa (JP); A63L/7088 (2006.01) Hidetoshi Ozawa, Kanagawa (JP); C07K 6/8 (2006.01) Leonard D. Shultz, Bar Harbor, C7H 2L/00 (2006.01) A6II 35/12 (2006.01) ME (US) CI2N 5/078 (2010.01) (52) U.S. Cl. .................. 424/173.1; 424/178.1; 424/93.7: (73) Assignee: RIKEN, Wako-shi (JP) 435/6.14; 435/723; 435/375; 506/9: 514/44 A: 530/389.6; 530/391.7:536/24.5 (57) ABSTRACT (21) Appl. No.: 13/258,993 The invention provides a test method for predicting the initial onset or a recurrence of acute myeloid leukemia (AML) com PCT Fled: prising (1) measuring the expression level of human leukemic (22) Mar. 24, 2010 stem cell (LSC) marker genes in a biological sample collected from a Subject for a transcription product or translation prod uct of the gene as an analyte and (2) comparing the expression (86) PCT NO.: PCT/UP2010/0551.31 level with a reference value; an LSC-targeting therapeutic agent for AML capable of Suppressing the expression of a S371 (c)(1), gene selected from among LSC marker genes or a Substance (2), (4) Date: Dec. -
The Mitochondrial Kinase PINK1 in Diabetic Kidney Disease
International Journal of Molecular Sciences Review The Mitochondrial Kinase PINK1 in Diabetic Kidney Disease Chunling Huang * , Ji Bian , Qinghua Cao, Xin-Ming Chen and Carol A. Pollock * Kolling Institute, Sydney Medical School, Royal North Shore Hospital, University of Sydney, St. Leonards, NSW 2065, Australia; [email protected] (J.B.); [email protected] (Q.C.); [email protected] (X.-M.C.) * Correspondence: [email protected] (C.H.); [email protected] (C.A.P.); Tel.: +61-2-9926-4784 (C.H.); +61-2-9926-4652 (C.A.P.) Abstract: Mitochondria are critical organelles that play a key role in cellular metabolism, survival, and homeostasis. Mitochondrial dysfunction has been implicated in the pathogenesis of diabetic kidney disease. The function of mitochondria is critically regulated by several mitochondrial protein kinases, including the phosphatase and tensin homolog (PTEN)-induced kinase 1 (PINK1). The focus of PINK1 research has been centered on neuronal diseases. Recent studies have revealed a close link between PINK1 and many other diseases including kidney diseases. This review will provide a concise summary of PINK1 and its regulation of mitochondrial function in health and disease. The physiological role of PINK1 in the major cells involved in diabetic kidney disease including proximal tubular cells and podocytes will also be summarized. Collectively, these studies suggested that targeting PINK1 may offer a promising alternative for the treatment of diabetic kidney disease. Keywords: PINK1; diabetic kidney disease; mitochondria; mitochondria quality control; mitophagy Citation: Huang, C.; Bian, J.; Cao, Q.; 1. Introduction Chen, X.-M.; Pollock, C.A. -
Molecular Genetic Delineation of 2Q37.3 Deletion in Autism and Osteodystrophy: Report of a Case and of New Markers for Deletion Screening by PCR
UC Irvine UC Irvine Previously Published Works Title Molecular genetic delineation of 2q37.3 deletion in autism and osteodystrophy: report of a case and of new markers for deletion screening by PCR. Permalink https://escholarship.org/uc/item/83f0x61r Journal Cytogenetics and cell genetics, 94(1-2) ISSN 0301-0171 Authors Smith, M Escamilla, JR Filipek, P et al. Publication Date 2001 DOI 10.1159/000048775 License https://creativecommons.org/licenses/by/4.0/ 4.0 Peer reviewed eScholarship.org Powered by the California Digital Library University of California Original Article Cytogenet Cell Genet 94:15–22 (2001) Molecular genetic delineation of 2q37.3 deletion in autism and osteodystrophy: report of a case and of new markers for deletion screening by PCR M. Smith, J.R. Escamilla, P. Filipek, M.E. Bocian, C. Modahl, P. Flodman, and M.A. Spence Department of Pediatrics, University of California, Irvine CA (USA) Abstract. We recently studied a patient who meets criteria us to determine the parental origin of the deletion in our for autistic disorder and has a 2q37 deletion. Molecular cyto- patient. DNA from 8–13 unrelated individuals was used to genetic studies were carried out using DNA isolated from 22 determine heterozygosity estimates for these markers. We re- different 2q37 mapped BACs to more precisely define the view four genes deleted in our patient – genes whose known extent of the chromosome deletion. We also analyzed 2q37 functions and sites of expression in the brain and/or bone make mapped polymorphic markers. In addition DNA sequences of them candidates for involvement in autism and/or the osteo- BACs in the deletion region were scanned to identify microsa- dystrophy observed in patients with 2q37.3 deletions. -
The C9orf72-Interacting Protein Smcr8 Is a Negative Regulator of Autoimmunity and Lysosomal Exocytosis
Downloaded from genesdev.cshlp.org on October 5, 2021 - Published by Cold Spring Harbor Laboratory Press The C9orf72-interacting protein Smcr8 is a negative regulator of autoimmunity and lysosomal exocytosis Yingying Zhang,1,2,3 Aaron Burberry,1,2,3 Jin-Yuan Wang,1,2,3 Jackson Sandoe,1,2,3 Sulagna Ghosh,1,2,3 Namrata D. Udeshi,4 Tanya Svinkina,4 Daniel A. Mordes,1,2,3,5 Joanie Mok,1,2,3 Maura Charlton,1,2,3 Quan-Zhen Li,6,7 Steven A. Carr,4 and Kevin Eggan1,2,3 1Department of Stem Cell and Regenerative Biology, 2Department of Molecular and Cellular Biology, Harvard University, Cambridge, Massachusetts 02138, USA; 3Stanley Center for Psychiatric Research, Broad Institute of Massachusetts Institute of Technology and Harvard, Cambridge, Massachusetts 02142, USA; 4Proteomics Platform, Broad Institute of MIT and Harvard, Cambridge, Massachusetts 02142, USA; 5Department of Pathology, Massachusetts General Hospital, Boston, Massachusetts 02114, USA; 6Department of Immunology, 7Department of Internal Medicine, University of Texas Southwestern Medical Center, Dallas, Texas 75390, USA While a mutation in C9ORF72 is the most common genetic contributor to amyotrophic lateral sclerosis (ALS), much remains to be learned concerning the function of the protein normally encoded at this locus. To elaborate further on functions for C9ORF72, we used quantitative mass spectrometry-based proteomics to identify interacting proteins in motor neurons and found that its long isoform complexes with and stabilizes SMCR8, which further enables interaction with WDR41. To study the organismal and cellular functions for this tripartite complex, we generated Smcr8 loss-of-function mutant mice and found that they developed phenotypes also observed in C9orf72 loss-of- function animals, including autoimmunity. -
A Computational Approach for Defining a Signature of Β-Cell Golgi Stress in Diabetes Mellitus
Page 1 of 781 Diabetes A Computational Approach for Defining a Signature of β-Cell Golgi Stress in Diabetes Mellitus Robert N. Bone1,6,7, Olufunmilola Oyebamiji2, Sayali Talware2, Sharmila Selvaraj2, Preethi Krishnan3,6, Farooq Syed1,6,7, Huanmei Wu2, Carmella Evans-Molina 1,3,4,5,6,7,8* Departments of 1Pediatrics, 3Medicine, 4Anatomy, Cell Biology & Physiology, 5Biochemistry & Molecular Biology, the 6Center for Diabetes & Metabolic Diseases, and the 7Herman B. Wells Center for Pediatric Research, Indiana University School of Medicine, Indianapolis, IN 46202; 2Department of BioHealth Informatics, Indiana University-Purdue University Indianapolis, Indianapolis, IN, 46202; 8Roudebush VA Medical Center, Indianapolis, IN 46202. *Corresponding Author(s): Carmella Evans-Molina, MD, PhD ([email protected]) Indiana University School of Medicine, 635 Barnhill Drive, MS 2031A, Indianapolis, IN 46202, Telephone: (317) 274-4145, Fax (317) 274-4107 Running Title: Golgi Stress Response in Diabetes Word Count: 4358 Number of Figures: 6 Keywords: Golgi apparatus stress, Islets, β cell, Type 1 diabetes, Type 2 diabetes 1 Diabetes Publish Ahead of Print, published online August 20, 2020 Diabetes Page 2 of 781 ABSTRACT The Golgi apparatus (GA) is an important site of insulin processing and granule maturation, but whether GA organelle dysfunction and GA stress are present in the diabetic β-cell has not been tested. We utilized an informatics-based approach to develop a transcriptional signature of β-cell GA stress using existing RNA sequencing and microarray datasets generated using human islets from donors with diabetes and islets where type 1(T1D) and type 2 diabetes (T2D) had been modeled ex vivo. To narrow our results to GA-specific genes, we applied a filter set of 1,030 genes accepted as GA associated. -
Drosophila and Human Transcriptomic Data Mining Provides Evidence for Therapeutic
Drosophila and human transcriptomic data mining provides evidence for therapeutic mechanism of pentylenetetrazole in Down syndrome Author Abhay Sharma Institute of Genomics and Integrative Biology Council of Scientific and Industrial Research Delhi University Campus, Mall Road Delhi 110007, India Tel: +91-11-27666156, Fax: +91-11-27662407 Email: [email protected] Nature Precedings : hdl:10101/npre.2010.4330.1 Posted 5 Apr 2010 Running head: Pentylenetetrazole mechanism in Down syndrome 1 Abstract Pentylenetetrazole (PTZ) has recently been found to ameliorate cognitive impairment in rodent models of Down syndrome (DS). The mechanism underlying PTZ’s therapeutic effect is however not clear. Microarray profiling has previously reported differential expression of genes in DS. No mammalian transcriptomic data on PTZ treatment however exists. Nevertheless, a Drosophila model inspired by rodent models of PTZ induced kindling plasticity has recently been described. Microarray profiling has shown PTZ’s downregulatory effect on gene expression in fly heads. In a comparative transcriptomics approach, I have analyzed the available microarray data in order to identify potential mechanism of PTZ action in DS. I find that transcriptomic correlates of chronic PTZ in Drosophila and DS counteract each other. A significant enrichment is observed between PTZ downregulated and DS upregulated genes, and a significant depletion between PTZ downregulated and DS dowwnregulated genes. Further, the common genes in PTZ Nature Precedings : hdl:10101/npre.2010.4330.1 Posted 5 Apr 2010 downregulated and DS upregulated sets show enrichment for MAP kinase pathway. My analysis suggests that downregulation of MAP kinase pathway may mediate therapeutic effect of PTZ in DS. Existing evidence implicating MAP kinase pathway in DS supports this observation. -
A New Synuclein-Transgenic Mouse Model for Early Parkinson's Reveals Molecular Features of Preclinical Disease
bioRxiv preprint doi: https://doi.org/10.1101/2020.04.04.016642; this version posted April 5, 2020. The copyright holder for this preprint (which was not certified by peer review) is the author/funder, who has granted bioRxiv a license to display the preprint in perpetuity. It is made available under aCC-BY-NC-ND 4.0 International license. A new synuclein-transgenic mouse model for early Parkinson's reveals molecular features of preclinical disease Diana M Hendrickx1,*,#, Pierre Garcia1,2,#, Amer Ashrafi1, Alessia Sciortino1, Kristopher J Schmit1, Heike Kollmus3, Nathalie Nicot4, Tony Kaoma5, Laurent Vallar6, Manuel Buttini1,*,$, Enrico Glaab1,$ 1 Luxembourg Centre for Systems Biomedicine (LCSB), University of Luxembourg, Belvaux, Luxembourg 2 Laboratoire National de Sant´e(LNS), Neuropathology Unit, Dudelange, Luxembourg 3 Department of Infection Genetics, Helmholtz Centre for Infection Research, Braunschweig, Germany 4 Quantitative Biology Unit, Luxembourg Institute of Health, Strassen, Luxembourg 5 Department of Oncology, Luxembourg Institute of Health, Strassen, Luxembourg 6 Genomics Research Unit, Luxembourg Institute of Health, Luxembourg, Luxembourg * [email protected]; [email protected] # equal contributor $ equal contributor Abstract Understanding Parkinson's disease (PD) in particular in its earliest phases is important for diagnosis and treatment. However, human brain samples are collected post-mortem, reflecting mainly end stage disease. Because brain samples of mouse models can be collected at any stage of the disease process, they are useful to investigate PD progression. Here, we compare ventral midbrain transcriptomics profiles from α-synuclein transgenic mice with a progressive, early PD-like striatum neurodegeneration across different ages using pathway, gene set and network analysis methods. -
C9orf72 Binds SMCR8, Localizes to Lysosomes and Regulates Mtorc1 Signaling
C9orf72 binds SMCR8, localizes to lysosomes and regulates mTORC1 signaling Joseph Amick1,2, Agnes Roczniak-Ferguson1,2 and Shawn M. Ferguson1,2* Department of Cell Biology1, Program in Cellular Neuroscience, Neurodegeneration and Repair2, Yale University School of Medicine, New Haven, CT, 06510 *To whom correspondence should be addressed e-mail: [email protected] phone: 203-737-5505 fax: 203-737-2065 Running Title: Lysosomal functions for C9orf72 Supplemental Material can be found at: 1 http://www.molbiolcell.org/content/suppl/2016/08/22/mbc.E16-01-0003v1.DC1.html Abstract Hexanucleotide expansion in an intron of the C9orf72 gene causes amyotrophic lateral sclerosis and frontotemporal dementia (ALS-FTD). However, beyond bioinformatics predictions that have suggested structural similarity to folliculin (FLCN), the Birt-Hogg-Dubé syndrome tumor suppressor, little is known about the normal functions of the C9orf72 protein. To address this problem, we used genome editing strategies to investigate C9orf72 interactions, subcellular localization and knockout (KO) phenotypes. We found that C9orf72 robustly interacts with SMCR8 (a protein of previously unknown function). We furthermore observed that C9orf72 localizes to lysosomes and that such localization is negatively regulated by amino acid availability. Analysis of C9orf72 KO, SMCR8 KO and double KO cell lines revealed phenotypes that are consistent with a function for C9orf72 at lysosomes. These include abnormally swollen lysosomes in the absence of C9orf72 as well as impaired responses of mTORC1 signaling to changes in amino acid availability (a lysosome-dependent process) following depletion of either C9orf72 or SMCR8. Collectively, these results identify strong physical and functional interactions between C9orf72 and SMCR8 and furthermore support a lysosomal site-of-action for this protein complex. -
Changes in Prostate Gene Expression in Men Undergoing an Intensive Nutrition and Lifestyle Intervention
Changes in prostate gene expression in men undergoing an intensive nutrition and lifestyle intervention Dean Ornish*†‡, Mark Jesus M. Magbanua§, Gerdi Weidner*, Vivian Weinberg¶, Colleen Kemp*, Christopher Green§, Michael D. Mattie§, Ruth Marlin*, Jeff Simkoʈ, Katsuto Shinohara§, Christopher M. Haqq§ and Peter R. Carroll§ §Department of Urology, The Helen Diller Family Comprehensive Cancer Center, and ʈDepartment of Pathology, University of California, 2340 Sutter Street, San Francisco, CA 94115; *Preventive Medicine Research Institute, 900 Bridgeway, Sausalito, CA 94965; †Department of Medicine, School of Medicine, University of California, 505 Parnassus Avenue, San Francisco, CA 94143; and ¶Biostatistics Core, The Helen Diller Family Comprehensive Cancer Center, University of California, 513 Parnassus Avenue, Box 0127, San Francisco, CA 94143 Communicated by J. Craig Venter, The J. Craig Venter Institute, Rockville, MD, April 2, 2008 (received for review February 13, 2008) Epidemiological and prospective studies indicate that comprehensive indolent low-risk prostate cancers, defined by strict clinical and lifestyle changes may modify the progression of prostate cancer. pathologic criteria designed to minimize the risk for metastatic However, the molecular mechanisms by which improvements in diet disease as a result of study participation (9). The 30 men who and lifestyle might affect the prostate microenvironment are poorly enrolled did not undergo surgery or radiation therapy to treat their understood. We conducted a pilot study to examine changes in low-risk tumors; rather, they underwent comprehensive lifestyle prostate gene expression in a unique population of men with low-risk changes (low-fat, whole-foods, plant-based nutrition; stress man- prostate cancer who declined immediate surgery, hormonal therapy, agement techniques; moderate exercise; and participation in a or radiation and participated in an intensive nutrition and lifestyle psychosocial group support). -
The Evi5 Family in Cellular Physiology and Pathology
FEBS Letters 587 (2013) 1703–1710 journal homepage: www.FEBSLetters.org Review The Evi5 family in cellular physiology and pathology ⇑ Yi Shan Lim a, Bor Luen Tang a,b, a Department of Biochemistry, Yong Loo Lin School of Medicine, National University Health System, Singapore b NUS Graduate School of Integrative Sciences and Engineering, National University of Singapore, 8 Medical Drive, Singapore 117597, Singapore article info abstract Article history: The Ecotropic viral integration site 5 (Evi5) and Evi5-like (Evi5L) belong to a small subfamily of the Received 25 February 2013 Tre-2/Bub2/Cdc16 (TBC) domain-containing proteins with enigmatically divergent roles as modula- Revised 23 April 2013 tors of cell cycle progression, cytokinesis, and cellular membrane traffic. First recognized as a poten- Accepted 28 April 2013 tial oncogene and a cell cycle regulator, Evi5 acts as a GTPase Activating Protein (GAP) for Rab11 in Available online 10 May 2013 cytokinesis. On the other hand, its homologue Evi5L has Rab-GAP activity towards Rab10 as well as Edited by Lukas Huber Rab23, and has been implicated in primary cilia formation. Recent genetic susceptibility analysis points to Evi5 as an important factor in susceptibility to multiple sclerosis. We discuss below the myriad of cellular functions exhibited by the Evi5 family members, and their associations with dis- Keywords: Cell cycle ease conditions. Evi5 Ó 2013 Federation of European Biochemical Societies. Published by Elsevier B.V. All rights reserved. GTPase Activating Protein (GAP) Rab -
Identifying Mitochondrial-Related Genes NDUFA10 and NDUFV2 As Prognostic Markers for Prostate Cancer Through Biclustering
Hindawi BioMed Research International Volume 2021, Article ID 5512624, 15 pages https://doi.org/10.1155/2021/5512624 Research Article Identifying Mitochondrial-Related Genes NDUFA10 and NDUFV2 as Prognostic Markers for Prostate Cancer through Biclustering Haokun Zhang ,1,2 Yuanhua Shao,1 Weijun Chen,1 and Xin Chen 1 1Guangdong Key Laboratory of IoT Information Technology, School of Automation, Guangdong University of Technology, Guangzhou 510006, China 2School of Computer Science and Engineering, Sun Yat-sen University, Guangzhou 510006, China Correspondence should be addressed to Xin Chen; [email protected] Received 15 February 2021; Accepted 5 May 2021; Published 24 May 2021 Academic Editor: Mohsin Khurshid Copyright © 2021 Haokun Zhang et al. This is an open access article distributed under the Creative Commons Attribution License, which permits unrestricted use, distribution, and reproduction in any medium, provided the original work is properly cited. Prostate cancer is currently associated with higher morbidity and mortality in men in the United States and Western Europe, so it is important to identify genes that regulate prostate cancer. The high-dimension gene expression profile impedes the discovery of biclusters which are of great significance to the identification of the basic cellular processes controlled by multiple genes and the identification of large-scale unknown effects hidden in the data. We applied the biclustering method MCbiclust to explore large biclusters in the TCGA cohort through a large number of iterations. Two biclusters were found with the highest silhouette coefficient value. The expression patterns of one bicluster are highly similar to those found by the gene expression profile of the known androgen-regulated genes. -
Skeletal Muscle Gene Expression in Long-Term Endurance and Resistance Trained Elderly
International Journal of Molecular Sciences Article Skeletal Muscle Gene Expression in Long-Term Endurance and Resistance Trained Elderly 1,2, 3, 1,2, Alessandra Bolotta y, Giuseppe Filardo y, Provvidenza Maria Abruzzo *, Annalisa Astolfi 4,5 , Paola De Sanctis 1, Alessandro Di Martino 6, Christian Hofer 7, Valentina Indio 4 , Helmut Kern 7, Stefan Löfler 7 , Maurilio Marcacci 8, Sandra Zampieri 9,10, 1,2, 1, Marina Marini z and Cinzia Zucchini z 1 Department of Experimental, Diagnostic and Specialty Medicine, University of Bologna School of Medicine, 40138 Bologna, Italy; [email protected] (A.B.); [email protected] (P.D.S.); [email protected] (M.M.); [email protected] (C.Z.) 2 IRCCS Fondazione Don Carlo Gnocchi, 20148 Milan, Italy 3 Applied and Translational Research Center, IRCCS Istituto Ortopedico Rizzoli, 40136 Bologna, Italy; g.fi[email protected] 4 Giorgio Prodi Interdepartimental Center for Cancer Research, S.Orsola-Malpighi Hospital, 40138 Bologna, Italy; annalisa.astolfi@unibo.it (A.A.); [email protected] (V.I.) 5 Department of Morphology, Surgery and Experimental Medicine, University of Ferrara, 44121 Ferrara, Italy 6 Second Orthopaedic and Traumatologic Clinic, IRCCS Istituto Ortopedico Rizzoli, 40136 Bologna, Italy; [email protected] 7 Ludwig Boltzmann Institute for Rehabilitation Research, 1160 Wien, Austria; [email protected] (C.H.); [email protected] (H.K.); stefan.loefl[email protected] (S.L.) 8 Department of Biomedical Sciences, Knee Joint Reconstruction Center, 3rd Orthopaedic Division, Humanitas Clinical Institute, Humanitas University, 20089 Milan, Italy; [email protected] 9 Department of Surgery, Oncology and Gastroenterology, University of Padua, 35122 Padua, Italy; [email protected] 10 Department of Biomedical Sciences, University of Padua, 35131 Padua, Italy * Correspondence: [email protected]; Tel.: +39-051-2094122 These authors contributed equally to this work.