Lyman Α Radiation Hydrodynamics of Galactic Winds Before Cosmic Reionization
Total Page:16
File Type:pdf, Size:1020Kb
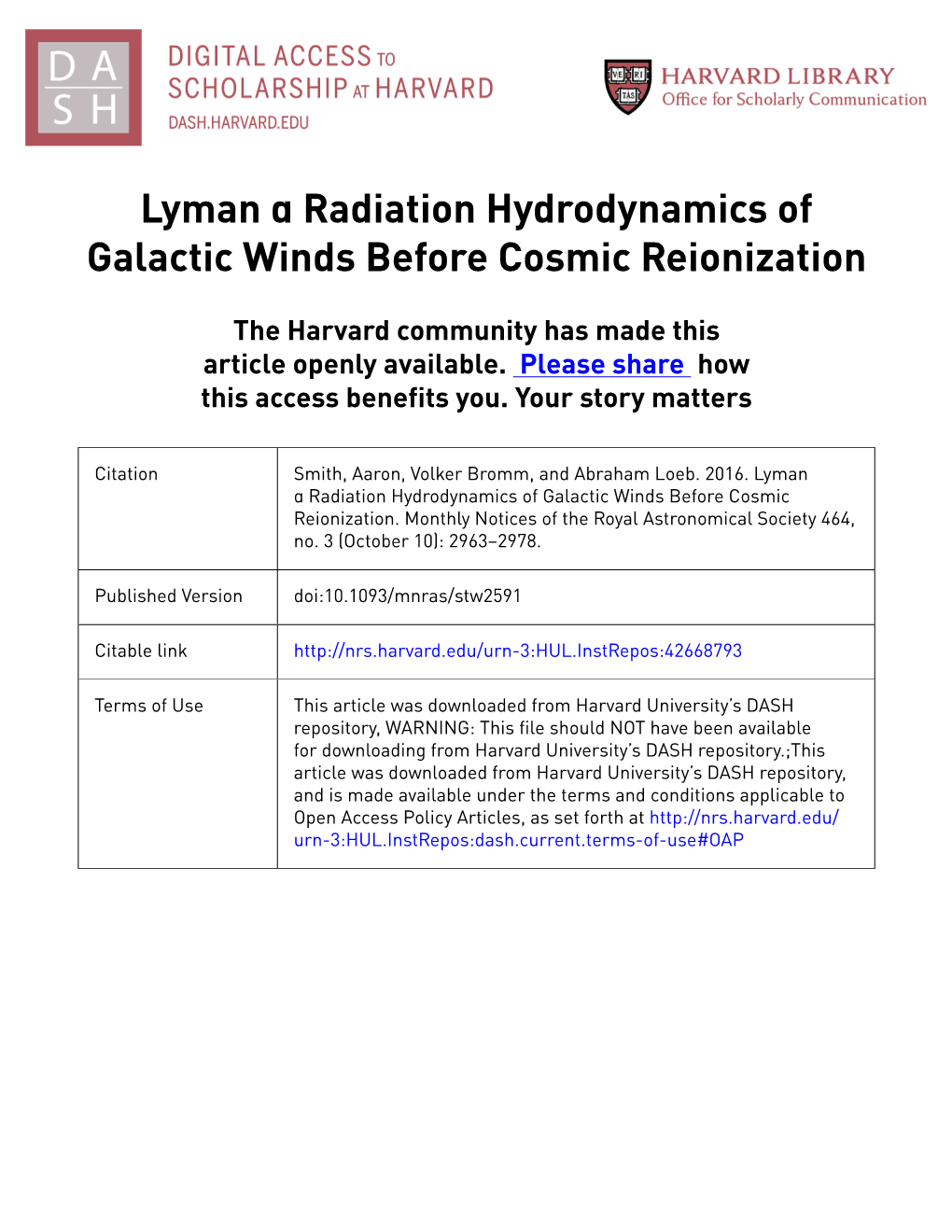
Load more
Recommended publications
-
Kepler-452B Is Not a New Earth a Twin of the Sun-Jupiter System
cover EN:l'astrofilo 28/08/15 10:12 Page 1 THE FREE MULTIMEDIA MAGAZINE THAT KEEPS YOU UPDATED ON WHAT IS HAPPENING IN SPACE Bi-monthly magazine of scientific and technical information ✶ September-October 2015 issue astonishing PLUTO Kepler-452b is not a new Earth A twinofthe Sun-Jupiter system www.astropublishing.com ✶ ita.astropublishing.com ✶ [email protected] colophon EN:l'astrofilo 28/08/15 10:17 Page 3 SUMMARY BI-MONTHLY MAGAZINE OF SCIENTIFIC AND TECHNICAL INFORMATION Astonishing Pluto FREELY AVAILABLE THROUGH A faultless mission, that of New Horizons: after a journey of nine and a half years it finally made its THE INTERNET rendezvous with Pluto one minute earlier than predicted and performed its task in the best of ways, 4 revealing a world remarkably more lively and varied than we could have imagined. In these pages... September-October 2015 Kepler-452b is not a new Earth Discovering a planet very similar to ours, at just the right distance from a star identical to the Sun, would be an important step forward in the search for extraterrestrial life. The rush to reach this goal 18 could, however, make us overly optimistic and lead us to define the same as Earth a planet that in... First detection of lithium from an exploding star The light chemical element lithium is one of the few elements that is predicted to have been created by the Big Bang, 13.8 billion years ago. But understanding the amounts of lithium observed in stars around 26 us today in the Universe has given astronomers headaches. -
A Multimessenger View of Galaxies and Quasars from Now to Mid-Century M
A multimessenger view of galaxies and quasars from now to mid-century M. D’Onofrio 1;∗, P. Marziani 2;∗ 1 Department of Physics & Astronomy, University of Padova, Padova, Italia 2 National Institute for Astrophysics (INAF), Padua Astronomical Observatory, Italy Correspondence*: Mauro D’Onofrio [email protected] ABSTRACT In the next 30 years, a new generation of space and ground-based telescopes will permit to obtain multi-frequency observations of faint sources and, for the first time in human history, to achieve a deep, almost synoptical monitoring of the whole sky. Gravitational wave observatories will detect a Universe of unseen black holes in the merging process over a broad spectrum of mass. Computing facilities will permit new high-resolution simulations with a deeper physical analysis of the main phenomena occurring at different scales. Given these development lines, we first sketch a panorama of the main instrumental developments expected in the next thirty years, dealing not only with electromagnetic radiation, but also from a multi-messenger perspective that includes gravitational waves, neutrinos, and cosmic rays. We then present how the new instrumentation will make it possible to foster advances in our present understanding of galaxies and quasars. We focus on selected scientific themes that are hotly debated today, in some cases advancing conjectures on the solution of major problems that may become solved in the next 30 years. Keywords: galaxy evolution – quasars – cosmology – supermassive black holes – black hole physics 1 INTRODUCTION: TOWARD MULTIMESSENGER ASTRONOMY The development of astronomy in the second half of the XXth century followed two major lines of improvement: the increase in light gathering power (i.e., the ability to detect fainter objects), and the extension of the frequency domain in the electromagnetic spectrum beyond the traditional optical domain. -
An Overview of New Worlds, New Horizons in Astronomy and Astrophysics About the National Academies
2020 VISION An Overview of New Worlds, New Horizons in Astronomy and Astrophysics About the National Academies The National Academies—comprising the National Academy of Sciences, the National Academy of Engineering, the Institute of Medicine, and the National Research Council—work together to enlist the nation’s top scientists, engineers, health professionals, and other experts to study specific issues in science, technology, and medicine that underlie many questions of national importance. The results of their deliberations have inspired some of the nation’s most significant and lasting efforts to improve the health, education, and welfare of the United States and have provided independent advice on issues that affect people’s lives worldwide. To learn more about the Academies’ activities, check the website at www.nationalacademies.org. Copyright 2011 by the National Academy of Sciences. All rights reserved. Printed in the United States of America This study was supported by Contract NNX08AN97G between the National Academy of Sciences and the National Aeronautics and Space Administration, Contract AST-0743899 between the National Academy of Sciences and the National Science Foundation, and Contract DE-FG02-08ER41542 between the National Academy of Sciences and the U.S. Department of Energy. Support for this study was also provided by the Vesto Slipher Fund. Any opinions, findings, conclusions, or recommendations expressed in this publication are those of the authors and do not necessarily reflect the views of the agencies that provided support for the project. 2020 VISION An Overview of New Worlds, New Horizons in Astronomy and Astrophysics Committee for a Decadal Survey of Astronomy and Astrophysics ROGER D. -
Mark Dijkstra
M. Dijkstra (UiO) Aspen, 2016 Mark Dijkstra with: Shiv Sethi (RRI), Abraham Loeb (CfA), Max Gronke (UiO) & David Sobral (Lancaster) arXiv:1601.04712 (ApJ accepted), Dijkstra, Sethi & Loeb arXiv:1602.07695 (resubmitted to ApJ), Dijkstra, Gronke & Sobral M. Dijkstra (UiO) Aspen, 2016 The Direct Collapse Black Hole Scenario `….as long as H2 formation is suppressed, these massive clumps do not fragment but rather cool and continue to collapse isothermally at a temperature of ~104 K.… ‘ from Bromm & Loeb 2003 Credit: John Wise M. Dijkstra (UiO) Aspen, 2016 The Direct Collapse Black Hole Scenario Extremely interesting from a [Lyα] radiative transfer (RT) point of view: 1. Pristine environment 2. Suppressed fragmentation 3. Suppressed H2 content. 4. No star formation & stellar feedback, dust. Ordinary challenges one faces when modelling interstellar Lyα RT are absent. Lyα `survival probability’ is maximised. M. Dijkstra (UiO) Aspen, 2016 Observational Signature of DCBHs I: Lyα Study Lyα RT through simplified models of DCBH formation. M. Dijkstra (UiO) Aspen, 2016 Observational Signature of DCBHs I: Lyα Study Lyα RT through simplified models of DCBH formation. M. Dijkstra (UiO) Aspen, 2016 Observational Signature of DCBHs I: Lyα Study Lyα RT through simplified models of DCBH formation. M. Dijkstra (UiO) Aspen, 2016 Results I: Lyα Luminosity Lyα luminosity as function of mean cloud density for cloud photoionized by central ionising source (powered by in place DCBH of mass MBH) fully ionised (density bound) cloud: recombination luminosity ~ n2 M. Dijkstra (UiO) Aspen, 2016 Results I: Lyα Luminosity Lyα luminosity as function of mean cloud density for cloud photoionized by central ionising source (powered by in place DCBH of mass MBH) Maximum luminosity set by black hole mass at ~ erg/s Independent of geometry! fesc —> 0 For predictions of emerging spectra, see Dijkstra, Gronke & Sobral 2016 M. -
The FIRST STARS in the UNIVERSE WERE TYPICALLY MANY TIMES More Massive and Luminous Than the Sun
THEFIRST STARS IN THE UNIVERSE Exceptionally massive and bright, the earliest stars changed the course of cosmic history We live in a universe that is full of bright objects. On a clear night one can see thousands of stars with the naked eye. These stars occupy mere- ly a small nearby part of the Milky Way galaxy; tele- scopes reveal a much vaster realm that shines with the light from billions of galaxies. According to our current understanding of cosmology, howev- er, the universe was featureless and dark for a long stretch of its early history. The first stars did not appear until perhaps 100 million years after the big bang, and nearly a billion years passed before galaxies proliferated across the cosmos. Astron- BY RICHARD B. LARSON AND VOLKER BROMM omers have long wondered: How did this dramat- ILLUSTRATIONS BY DON DIXON ic transition from darkness to light come about? 4 SCIENTIFIC AMERICAN Updated from the December 2001 issue COPYRIGHT 2004 SCIENTIFIC AMERICAN, INC. EARLIEST COSMIC STRUCTURE mmostost likely took the form of a network of filaments. The first protogalaxies, small-scale systems about 30 to 100 light-years across, coalesced at the nodes of this network. Inside the protogalaxies, the denser regions of gas collapsed to form the first stars (inset). COPYRIGHT 2004 SCIENTIFIC AMERICAN, INC. After decades of study, researchers The Dark Ages to longer wavelengths and the universe have recently made great strides toward THE STUDY of the early universe is grew increasingly cold and dark. As- answering this question. Using sophisti- hampered by a lack of direct observa- tronomers have no observations of this cated computer simulation techniques, tions. -
15.1 Galaxy Formation: Introduction
15.1 Galaxy Formation: Introduction Galaxy Formation • The early stages of galaxy evolution - but there is no clear-cut boundary, and it also has two principal aspects: assembly of the mass, and conversion of gas into stars • Must be related to large-scale (hierarchical) structure formation, plus the dissipative processes - it is a very messy process, much more complicated than LSS formation and growth • Probably closely related to the formation of the massive central black holes as well • Generally, we think of massive galaxy formation at high redshifts (z ~ 3 - 10, say); dwarfs may be still forming now • Observations have found populations of what must be young galaxies (ages < 1 Gyr), ostensibly progenitors of large galaxies today, at z ~ 5 - 7 • The frontier is now at z ~ 7 - 20, the so-called Reionization Era 2 A General Outline • The smallest scale density fluctuations keep collapsing, with baryons falling into the potential wells dominated by the dark matter, achieving high densties through cooling – This process starts right after the recombination at z ~ 1100 • Once the gas densities are high enough, star formation ignites – This probably happens around z ~ 20 - 30 – By z ~ 6, UV radiation from young galaxies reionizes the unverse • These protogalactic fragments keep merging, forming larger objects in a hierarchical fashion ever since then • Star formation enriches the gas, and some of it is expelled in the intergalactic medium, while more gas keeps falling in • If a central massive black hole forms, the energy release from accretion -
Second Parameter Globulars and Dwarf Spheroidals Around the Local Group Massive Galaxies: What Can They Evidence?
A&A 396, 117–123 (2002) Astronomy DOI: 10.1051/0004-6361:20021404 & c ESO 2002 Astrophysics Second parameter globulars and dwarf spheroidals around the Local Group massive galaxies: What can they evidence? V. K ravtsov Sternberg Astronomical Institute, University Avenue 13, 119899 Moscow, Russia Received 29 April 2002 / Accepted 28 August 2002 Abstract. We suggest that the majority of the “young”, so–called “second parameter” globular clusters (SPGCs) have origi- nated in the outer Galactic halo due to a process other than a tidal disruption of the dwarf spheroidal (dSph) galaxies. Basic observational evidence regarding both the dSphs and the SPGCs, coupled with the latest data about a rather large relative num- ber of such clusters among globulars in M 33 and their low portion in M 31, seems to be consistent with the suspected process. It might have taken place within the system of the most massive galaxies of the Local Group (LG) at the earliest stages of their formation and evolution. We argue that the origin and basic characteristics of the SPGCs can naturally be explained as a result of mass outflow from M 31, during and due to formation of its Pop. II stars, and subsequent accretion of gas onto its massive companions, the Galaxy and M 33. An amount of the gas accreted onto the Milky Way is expected to have been quite enough for the formation in the outer Galactic halo not only of the clusters under consideration but also a number of those dSph galaxies which are as young as the SPGCs. A less significant, but notable mass transfer from the starbursting protoGalaxy to the massive members of the LG might have occurred, too. -
Formation and Growth of the First Supermassive Black Holes
Formation and Growth of the First Supermassive Black Holes Ph.D. Thesis by Tilman Hartwig UNIVERSITÉ PIERRE ET MARIE CURIE École Doctorale d’Astronomie et Astrophysique d’Île-de-France Ph.D. Thesis to obtain the title of Doctor in Astrophysics of the University Pierre et Marie Curie Presented by Tilman Hartwig Formation and Growth of the First Supermassive Black Holes Thesis Advisor: Dr. Marta Volonteri prepared at the Institut d’Astrophysique de Paris, CNRS (UMR 7095), Université Pierre et Marie Curie (Paris VI) with financial support from the European Research Council grant ‘BLACK’ Presented and publicly defended on the 22nd of September, 2017 to a jury consisting of Reviewers: Michela Mapelli - INAF, Padova, Italy Jarrett Johnson - LANL, Los Alamos, USA Advisor: Marta Volonteri - IAP, Paris, France President: Frédéric Daigne - IAP, Paris, France Examiner: Ralf Klessen - ZAH, Heidelberg, Germany Formation and Growth of the First Supermassive Black Holes Supermassive black holes reside in the centres of most massive galaxies and we observe correlations between their mass and properties of the host galaxies, such as bulge mass or stellar velocity dispersion. Besides this correlation between a galaxy and its central black hole (BH), we see BHs more massive than one billion solar masses already a few hundred million years after the Big Bang. These supermassive BHs at high redshift are just the tip of the iceberg of the entire BH population, but they challenge our understanding of the formation and growth of the first BHs. With this thesis, I contribute to a better understanding of the formation and growth of the first supermassive BHs with theoretical models and comparisons to recent observations. -
The First Galaxies
First Galaxies 1 The First Galaxies Volker Bromm1 and Naoki Yoshida2 1 Department of Astronomy and Texas Cosmology Center, University of Texas, Austin, Texas 78712; email: [email protected] 2 Institute for the Physics and Mathematics of the Universe, University of Tokyo, Kashiwa, Chiba 277-8583, Japan; email: [email protected] Key Words cosmology, galaxy formation, intergalactic medium, star formation, Population III Abstract We review our current understanding of how the first galaxies formed at the end of the cosmic dark ages, a few 100 million years after the Big Bang. Modern large telescopes discovered galaxies at redshifts greater than seven, whereas theoretical studies have just reached the degree of sophistication necessary to make meaningful predictions. A crucial ingredient is the feedback exerted by the first generation of stars, through UV radiation, supernova blast waves, and chemical enrichment. The key goal is to derive the signature of the first galaxies to be observed with upcoming or planned next-generation facilities, such as the James Webb Space Telescope or Atacama Large Millimeter Array. ¿From the observational side, ongoing deep-field searches for very high-redshift galaxies begin to provide us with empirical constraints on the nature of the first galaxies. CONTENTS INTRODUCTION .................................... 2 Annu. Rev. Astron. Astrophys. 2011 49 1056-8700/97/0610-00 WHAT IS A FIRST GALAXY? ............................ 6 Theoretical Perspective .................................... 7 Observational -
The Never-Ending Realm of Galaxies
The Multi-Bang Universe: The Never-Ending Realm of Galaxies Mário Everaldo de Souza Departamento de Física, Universidade Federal de Sergipe, 49.100-000, Brazil Abstract A new cosmological model is proposed for the dynamics of the Universe and the formation and evolution of galaxies. It is shown that the matter of the Universe contracts and expands in cycles, and that galaxies in a particu- lar cycle have imprints from the previous cycle. It is proposed that RHIC’s liquid gets trapped in the cores of galaxies in the beginning of each cycle and is liberated with time and is, thus, the power engine of AGNs. It is also shown that the large-scale structure is a permanent property of the Universe, and thus, it is not created. It is proposed that spiral galaxies and elliptical galaxies are formed by mergers of nucleon vortices (vorteons) at the time of the big squeeze and immediately afterwards and that the merging process, in general, lasts an extremely long time, of many billion years. It is concluded then that the Universe is eternal and that space should be infinite or almost. Keywords Big Bang, RHIC’s liquid, Galaxy Formation, Galaxy Evolution, LCDM Bang Theory: the universal expansion, the Cosmic Micro- 1. Introduction wave Background (CMB) radiation and Primordial or Big The article from 1924 by Alexander Friedmann "Über Bang Nucleosynthesis (BBN). Since then there have been die Möglichkeit einer Welt mit konstanter negativer several improvements in the measurements of the CMB Krümmung des Raumes" ("On the possibility of a world spectrum and its anisotropies by COBE [6], WMAP [7], with constant negative curvature of space") is the theoreti- and Planck 2013 [8]. -
8 October Version
A F irst T ransients Survey with JWST: the FLARE project Lifan Wang et al 8 October version Received ; accepted –2– ABSTRACT JWST was conceived and built to answer one of the most fundamental ques- tions that humans can address empirically: “How did the Universe make its first stars?”. This can be attempted in classical stare mode and by still photography - with all the pitfalls of crowding and multi-band redshifts of objects of which a spectrum was never obtained. Our First Lights At REionization (FLARE) project transforms the quest for the epoch of reionization from the static to the time domain. It targets the complementary question: “What happened to those first stars?”. It will be answered by observations of the most luminous events: supernovae and accretion on to black holes formed by direct collapse from the primordial gas clouds. These transients provide direct constraints on star-formation rates (SFRs) and the truly initial Initial Mass Function (IMF), and they may identify possible stellar seeds of supermassive black holes (SMBHs). Furthermore, our knowledge of the physics of these events at ultra-low metallic- ity will be much expanded. JWST’s unique capabilities will detect these most luminous and earliest cosmic messengers easily in fairly shallow observations. However, these events are very rare at the dawn of cosmic structure formation and so require large area coverage. Time domain astronomy can be advanced to an unprecedented depth by means of a shallow field of JWST reaching 27 mag AB in 2 µm and 4.4 µm over a field as large as 0.1 square degree visited multiple times each year. -
Baseline Metal Enrichment from Population III Star Formation in Cosmological Volume Simulations
MNRAS 000,1{ ?? (2017) Preprint 19 January 2018 Compiled using MNRAS LATEX style file v3.0 Baseline Metal Enrichment from Population III Star Formation in Cosmological Volume Simulations Jason Jaacks1?, Robert Thompson2;3, Steven L. Finkelstein1 and Volker Bromm1 1 Department of Astronomy, The University of Texas at Austin, Austin, TX 78712 2 Portalarium Inc., Austin, TX 78731 3 National Center for Supercomputing Applications, University of Illinois at Urbana-Champaign, Champaign, IL 61801 Accepted XXX. Received YYY; in original form ZZZ ABSTRACT We utilize the hydrodynamic and N-body code GIZMO coupled with our newly devel- oped sub-grid Population III (Pop III) Legacy model, designed specifically for cosmo- logical volume simulations, to study the baseline metal enrichment from Pop III star formation at z > 7. In this idealized numerical experiment, we only consider Pop III star formation. We find that our model Pop III star formation rate density (SFRD), −3 −1 −1 which peaks at ∼ 10 M yr Mpc near z ∼ 10, agrees well with previous numerical studies and is consistent with the observed estimates for Pop II SFRDs. The mean Pop III metallicity rises smoothly from z = 25 − 7, but does not reach the critical −4 metallicity value, Zcrit = 10 Z , required for the Pop III to Pop II transition in star formation mode until z ' 7. This suggests that, while individual halos can suppress in-situ Pop III star formation, the external enrichment is insufficient to globally ter- minate Pop III star formation. The maximum enrichment from Pop III star formation −2 in star forming dark matter halos is Z ∼ 10 Z , whereas the minimum found in −7 externally enriched haloes is Z & 10 Z .