Toward High-Spatial Resolution Gravity Surveying of the Mid-Ocean Ridges with Autonomous Underwater Vehicles James C
Total Page:16
File Type:pdf, Size:1020Kb
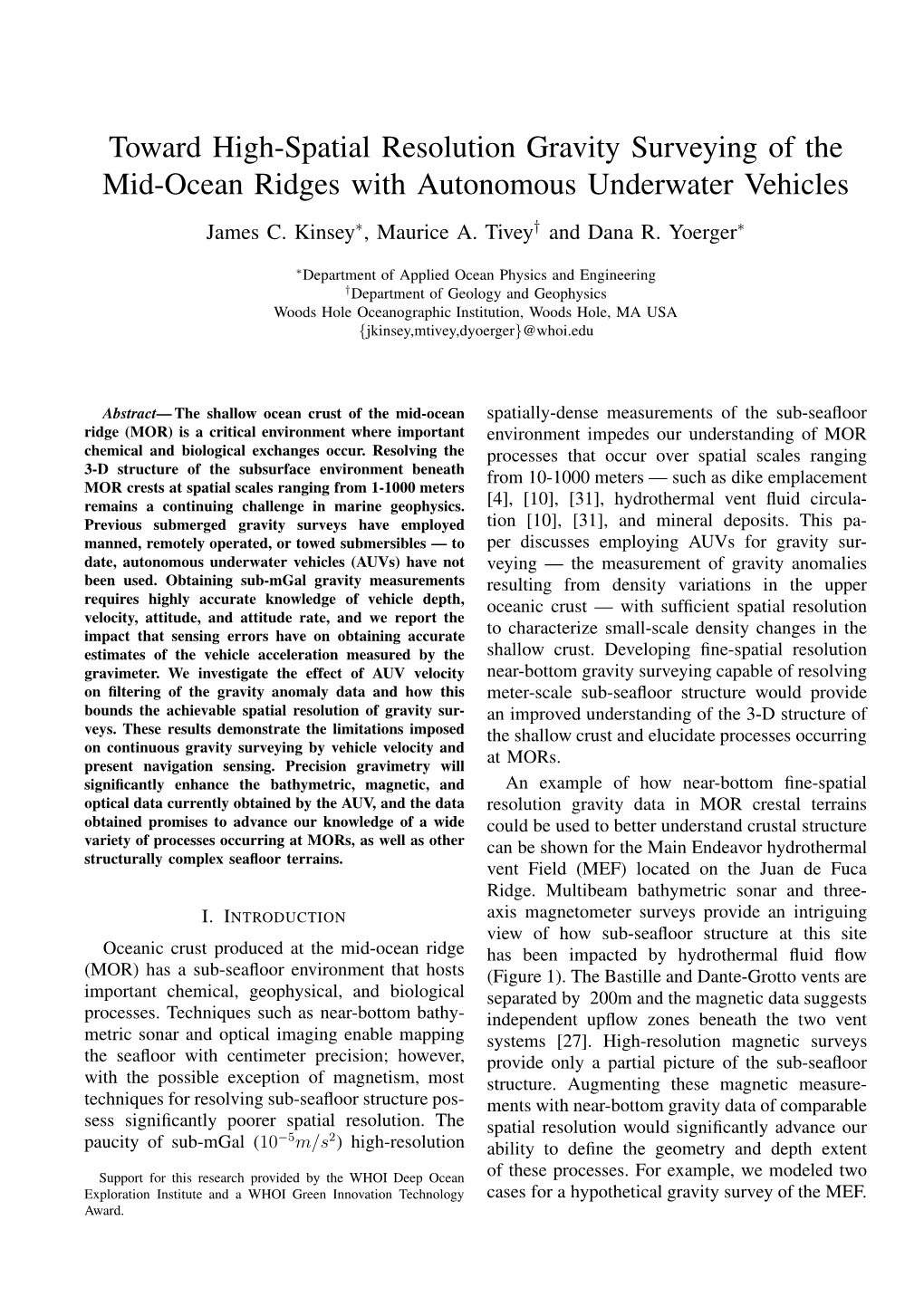
Load more
Recommended publications
-
Preliminary Estimation and Validation of Polar Motion Excitation from Different Types of the GRACE and GRACE Follow-On Missions Data
remote sensing Article Preliminary Estimation and Validation of Polar Motion Excitation from Different Types of the GRACE and GRACE Follow-On Missions Data Justyna Sliwi´ ´nska 1,* , Małgorzata Wi ´nska 2 and Jolanta Nastula 1 1 Space Research Centre, Polish Academy of Sciences, 00-716 Warsaw, Poland; [email protected] 2 Faculty of Civil Engineering, Warsaw University of Technology, 00-637 Warsaw, Poland; [email protected] * Correspondence: [email protected] Received: 18 September 2020; Accepted: 22 October 2020; Published: 23 October 2020 Abstract: The Gravity Recovery and Climate Experiment (GRACE) mission has provided global observations of temporal variations in the gravity field resulting from mass redistribution at the surface and within the Earth for the period 2002–2017. Although GRACE satellites are not able to realistically detect the second zonal parameter (DC20) of geopotential associated with the flattening of the Earth, they can accurately determine variations in degree-2 order-1 (DC21, DS21) coefficients that are proportional to variations in polar motion. Therefore, GRACE measurements are commonly exploited to interpret polar motion changes due to variations in the global mass redistribution, especially in the continental hydrosphere and cryosphere. Such impacts are usually examined by computing the so-called hydrological polar motion excitation (HAM) and cryospheric polar motion excitation (CAM), often analyzed together as HAM/CAM. The great success of the GRACE mission and the scientific robustness of its data contributed to the launch of its successor, GRACE Follow-On (GRACE-FO), which began in May 2018 and continues to the present. This study presents the first estimates of HAM/CAM computed from GRACE-FO data provided by three data centers: Center for Space Research (CSR), Jet Propulsion Laboratory (JPL), and GeoForschungsZentrum (GFZ). -
A Fast Method for Calculation of Marine Gravity Anomaly
applied sciences Article A Fast Method for Calculation of Marine Gravity Anomaly Yuan Fang 1, Shuiyuan He 2,*, Xiaohong Meng 1,*, Jun Wang 1, Yongkang Gan 1 and Hanhan Tang 1 1 School of Geophysics and Information Technology, China University of Geosciences, Beijing 100083, China; [email protected] (Y.F.); [email protected] (J.W.); [email protected] (Y.G.); [email protected] (H.T.) 2 Guangzhou Marine Geological Survey, China Geological Survey, Ministry of Land and Resources, Guangzhou 510760, China * Correspondence: [email protected] (S.H.); [email protected] (X.M.); Tel.: +86-136-2285-1110 (S.H.); +86-136-9129-3267 (X.M.) Abstract: Gravity data have been playing an important role in marine exploration and research. However, obtaining gravity data over an extensive marine area is expensive and inefficient. In reality, marine gravity anomalies are usually calculated from satellite altimetry data. Over the years, numer- ous methods have been presented for achieving this purpose, most of which are time-consuming due to the integral calculation over a global region and the singularity problem. This paper proposes a fast method for the calculation of marine gravity anomalies. The proposed method introduces a novel scheme to solve the singularity problem and implements the parallel technique based on a graphics processing unit (GPU) for fast calculation. The details for the implementation of the proposed method are described, and it is tested using the geoid height undulation from the Earth Gravitational Model 2008 (EGM2008). The accuracy of the presented method is evaluated by comparing it with marine shipboard gravity data. -
THE EARTH's GRAVITY OUTLINE the Earth's Gravitational Field
GEOPHYSICS (08/430/0012) THE EARTH'S GRAVITY OUTLINE The Earth's gravitational field 2 Newton's law of gravitation: Fgrav = GMm=r ; Gravitational field = gravitational acceleration g; gravitational potential, equipotential surfaces. g for a non–rotating spherically symmetric Earth; Effects of rotation and ellipticity – variation with latitude, the reference ellipsoid and International Gravity Formula; Effects of elevation and topography, intervening rock, density inhomogeneities, tides. The geoid: equipotential mean–sea–level surface on which g = IGF value. Gravity surveys Measurement: gravity units, gravimeters, survey procedures; the geoid; satellite altimetry. Gravity corrections – latitude, elevation, Bouguer, terrain, drift; Interpretation of gravity anomalies: regional–residual separation; regional variations and deep (crust, mantle) structure; local variations and shallow density anomalies; Examples of Bouguer gravity anomalies. Isostasy Mechanism: level of compensation; Pratt and Airy models; mountain roots; Isostasy and free–air gravity, examples of isostatic balance and isostatic anomalies. Background reading: Fowler §5.1–5.6; Lowrie §2.2–2.6; Kearey & Vine §2.11. GEOPHYSICS (08/430/0012) THE EARTH'S GRAVITY FIELD Newton's law of gravitation is: ¯ GMm F = r2 11 2 2 1 3 2 where the Gravitational Constant G = 6:673 10− Nm kg− (kg− m s− ). ¢ The field strength of the Earth's gravitational field is defined as the gravitational force acting on unit mass. From Newton's third¯ law of mechanics, F = ma, it follows that gravitational force per unit mass = gravitational acceleration g. g is approximately 9:8m/s2 at the surface of the Earth. A related concept is gravitational potential: the gravitational potential V at a point P is the work done against gravity in ¯ P bringing unit mass from infinity to P. -
Detection of Caves by Gravimetry
Detection of Caves by Gravimetry By HAnlUi'DO J. Cmco1) lVi/h plates 18 (1)-21 (4) Illtroduction A growing interest in locating caves - largely among non-speleolo- gists - has developed within the last decade, arising from industrial or military needs, such as: (1) analyzing subsUl'face characteristics for building sites or highway projects in karst areas; (2) locating shallow caves under airport runways constructed on karst terrain covered by a thin residual soil; and (3) finding strategic shelters of tactical significance. As a result, geologists and geophysicists have been experimenting with the possibility of applying standard geophysical methods toward void detection at shallow depths. Pioneering work along this line was accomplishecl by the U.S. Geological Survey illilitary Geology teams dUl'ing World War II on Okinawan airfields. Nicol (1951) reported that the residual soil covel' of these runways frequently indicated subsi- dence due to the collapse of the rooves of caves in an underlying coralline-limestone formation (partially detected by seismic methods). In spite of the wide application of geophysics to exploration, not much has been published regarding subsUl'face interpretation of ground conditions within the upper 50 feet of the earth's surface. Recently, however, Homberg (1962) and Colley (1962) did report some encoUl'ag- ing data using the gravity technique for void detection. This led to the present field study into the practical means of how this complex method can be simplified, and to a use-and-limitations appraisal of gravimetric techniques for speleologic research. Principles all(1 Correctiolls The fundamentals of gravimetry are based on the fact that natUl'al 01' artificial voids within the earth's sUl'face - which are filled with ail' 3 (negligible density) 01' water (density about 1 gmjcm ) - have a remark- able density contrast with the sUl'roun<ling rocks (density 2.0 to 1) 4609 Keswick Hoad, Baltimore 10, Maryland, U.S.A. -
Airborne Geoid Determination
LETTER Earth Planets Space, 52, 863–866, 2000 Airborne geoid determination R. Forsberg1, A. Olesen1, L. Bastos2, A. Gidskehaug3,U.Meyer4∗, and L. Timmen5 1KMS, Geodynamics Department, Rentemestervej 8, 2400 Copenhagen NV, Denmark 2Astronomical Observatory, University of Porto, Portugal 3Institute of Solid Earth Physics, University of Bergen, Norway 4Alfred Wegener Institute, Bremerhaven, Germany 5Geo Forschungs Zentrum, Potsdam, Germany (Received January 17, 2000; Revised August 18, 2000; Accepted August 18, 2000) Airborne geoid mapping techniques may provide the opportunity to improve the geoid over vast areas of the Earth, such as polar areas, tropical jungles and mountainous areas, and provide an accurate “seam-less” geoid model across most coastal regions. Determination of the geoid by airborne methods relies on the development of airborne gravimetry, which in turn is dependent on developments in kinematic GPS. Routine accuracy of airborne gravimetry are now at the 2 mGal level, which may translate into 5–10 cm geoid accuracy on regional scales. The error behaviour of airborne gravimetry is well-suited for geoid determination, with high-frequency survey and downward continuation noise being offset by the low-pass gravity to geoid filtering operation. In the paper the basic principles of airborne geoid determination are outlined, and examples of results of recent airborne gravity and geoid surveys in the North Sea and Greenland are given. 1. Introduction the sea-surface (H) by airborne altimetry. This allows— Precise geoid determination has in recent years been facil- in principle—the determination of the dynamic sea-surface itated through the progress in airborne gravimetry. The first topography (ζ) through the equation large-scale aerogravity experiment was the airborne gravity survey of Greenland 1991–92 (Brozena, 1991). -
Gravity Anomaly Measuring Gravity
Gravity Newton’s Law of Gravity (1665) 2 F = G (m1m2) / r F = force of gravitational attraction m1 and m2 = mass of 2 attracting objects r = distance between the two objects G -- ? Earth dimensions: 3 rearth = 6.378139 x 10 km (equator) 27 mearth = 5.976 x 10 g 2 F = G (m1m2) / r ) 2 F = mtest (G mEarth / rEarth ) Newton’s Second Law: F = ma implies that the acceleration at the surface of the Earth is: 2 (G mEarth / rEarth ) = ~ 981 cm/sec2 = 981 “Gals” variations are on the order of (0.1 mgal) Precision requires 2 spatially based corrections must account for 2 facts about the Earth it is not round (.... it’s flattened at the poles) it is not stationary ( .... it’s spinning) Earth is an ellipsoid first detected by Newton in 1687 clocks 2 min/day slower at equator than in England concluded regional change in "g" controlling pendulum translated this 2 min into different values of Earth radii radiusequator > radiuspole requator / rpole = 6378/6357 km = flattening of ~1/298 two consequences equator farther from center than poles hence gravity is 6.6 Gals LESS at the equator equator has more mass near it than do the poles hence gravity is 4.8 Gals MORE at the equator result gravity is 1.8 Gals LESS at the equator Earth is a rotating object circumference at equator ~ 40,000 km; at poles 0 km all parts of planet revolve about axis once in 24 hrs hence equator spins at 40,000 =1667 km/hr; at poles 0 km/hr 24 outward centrifugal force at equator; at poles 0 result .... -
Resolving Geophysical Signals by Terrestrial Gravimetry: a Time Domain Assessment of the Correction-Induced Uncertainty
Originally published as: Mikolaj, M., Reich, M., Güntner, A. (2019): Resolving Geophysical Signals by Terrestrial Gravimetry: A Time Domain Assessment of the Correction-Induced Uncertainty. - Journal of Geophysical Research, 124, 2, pp. 2153—2165. DOI: http://doi.org/10.1029/2018JB016682 RESEARCH ARTICLE Resolving Geophysical Signals by Terrestrial Gravimetry: 10.1029/2018JB016682 A Time Domain Assessment of the Correction-Induced Key Points: Uncertainty • Global-scale uncertainty assessment of tidal, oceanic, large-scale 1 1 1,2 hydrological, and atmospheric M. Mikolaj , M. Reich , and A. Güntner corrections for terrestrial gravimetry • Resolving subtle gravity signals in the 1Section Hydrology, GFZ German Research Centre for Geosciences, Potsdam, Germany, 2Institute of Environmental order of few nanometers per square Science and Geography, University of Potsdam, Potsdam, Germany second is challenged by the statistical uncertainty of correction models • Uncertainty computed for selected periods varies significantly Abstract Terrestrial gravimetry is increasingly used to monitor mass transport processes in with latitude and altitude of the geophysics boosted by the ongoing technological development of instruments. Resolving a particular gravimeter phenomenon of interest, however, requires a set of gravity corrections of which the uncertainties have not been addressed up to now. In this study, we quantify the time domain uncertainty of tide, global Supporting Information: atmospheric, large-scale hydrological, and nontidal ocean loading corrections. The uncertainty is • Supporting Information S1 assessed by comparing the majority of available global models for a suite of sites worldwide. The average uncertainty expressed as root-mean-square error equals 5.1 nm/s2, discounting local hydrology or air Correspondence to: pressure. The correction-induced uncertainty of gravity changes over various time periods of interest M. -
Macroscopic Superpositions and Gravimetry with Quantum Magnetomechanics Received: 07 July 2016 Mattias T
www.nature.com/scientificreports OPEN Macroscopic superpositions and gravimetry with quantum magnetomechanics Received: 07 July 2016 Mattias T. Johnsson, Gavin K. Brennen & Jason Twamley Accepted: 24 October 2016 Precision measurements of gravity can provide tests of fundamental physics and are of broad Published: 21 November 2016 practical interest for metrology. We propose a scheme for absolute gravimetry using a quantum magnetomechanical system consisting of a magnetically trapped superconducting resonator whose motion is controlled and measured by a nearby RF-SQUID or flux qubit. By driving the mechanical massive resonator to be in a macroscopic superposition of two different heights our we predict that our interferometry protocol could, subject to systematic errors, achieve a gravimetric sensitivity of Δg/g ~ 2.2 × 10−10 Hz−1/2, with a spatial resolution of a few nanometres. This sensitivity and spatial resolution exceeds the precision of current state of the art atom-interferometric and corner-cube gravimeters by more than an order of magnitude, and unlike classical superconducting interferometers produces an absolute rather than relative measurement of gravity. In addition, our scheme takes measurements at ~10 kHz, a region where the ambient vibrational noise spectrum is heavily suppressed compared the ~10 Hz region relevant for current cold atom gravimeters. Gravimetry is the measurement of the local acceleration due to gravity on a test body. As gravity is an infinite range force that cannot be shielded against, it provides a useful probe for a variety of effects over a wide range of distances. Given a theory of gravity, gravimetry provides information about local mass distribution or, given a known, local mass distribution, it provides information that can be used test theories of gravity. -
Interpreting Gravity Anomalies in South Cameroon, Central Africa
EARTH SCIENCES RESEARCH JOURNAL Earth Sci Res SJ Vol 16, No 1 (June, 2012): 5 - 9 GRAVITY EXPLORATION METHODS Interpreting gravity anomalies in south Cameroon, central Africa Yves Shandini1 and Jean Marie Tadjou2* 1 Physics Department, Science Faculty, University of Yaoundé I, Cameroon 2 National Institute of Cartography- NIC, Yaoundé, Cameroon * Corresponding author E-mail: shandiniyves@yahoo fr Abstract Keywords: fault, gravity data, isostatic residual gravity, south Cameroon, total horizontal derivative The area involved in this study is the northern part of the Congo craton, located in south Cameroon, (2 5°N - 4 5°N, 11°E - 13°E) The study involved analysing gravity data to delineate major structures and faults in south Cameroon The region’s Bouguer gravity is characterised by elongated SW-NE negative gravity anomaly corresponding to a collapsed structure associated with a granitic intrusion beneath the region, limited by fault systems; this was clearly evident on an isostatic residual gravity map High grav- ity anomaly within the northern part of the area was interpreted as a result of dense bodies put in place at the root of the crust Positive anomalies in the northern part of the area were separated from southern negative anomalies by a prominent E-W lineament; this was interpreted on the gravity maps as a suture zone between the south Congo craton and the Pan-African formations Gravity anomalies’ total horizontal derivatives generally reflect faults or compositional changes which can describe structural trends The lo- cal maxima -
Pendulum MEMS Gravimeters for Semi-Absolute Gravimetry
EGU2020-1244 https://doi.org/10.5194/egusphere-egu2020-1244 EGU General Assembly 2020 © Author(s) 2021. This work is distributed under the Creative Commons Attribution 4.0 License. Pendulum MEMS gravimeters for semi-absolute gravimetry Richard Middlemiss1, Giles Hammond2, Richard Walker2, and Abhinav Prasad2 1University of Glasgow, James Watt School of Engineering, Glasgow, United Kingdom of Great Britain and Northern Ireland ([email protected]) 2University of Glasgow, Institute for Gravitational Research, Glasgow, United Kingdom of Great Britain and Northern Ireland By measuring tiny variations in the Earth’s gravitational acceleration, g, one can infer density variations beneath the ground. Since magmatic systems contain rock of differing density, changes in gravity over time can tell us when/where magma is moving. Traditional gravity sensors (gravimeters) were costly and heavy, but with the advent of the technology used to make mobile phone accelerometers (MEMS – Microelectromechanical-systems), this is changing. At Glasgow University we have already developed the first MEMS gravity sensor and we are now working with several other European institutions to make a network of gravity sensors around Mt Etna – NEWTON-g. It will be the first multi-pixel gravity imager – enabling unprecedented resolution of Etna’s plumbing system. While this work is ongoing, a second generation of MEMS gravity sensor is now under development. The first-generation sensor comprises a mass on a spring, which moves in response to changing values of g. This, however, can only ever be used to measure changes in gravity, which means it can be difficult to tell the difference between a geophysical signal and instrumental drift. -
Gravimetric Geoid Modelling Over Peninsular Malaysia Using Two Different Gridding Approaches for Combining Free Air Anomaly
The International Archives of the Photogrammetry, Remote Sensing and Spatial Information Sciences, Volume XLII-4/W16, 2019 6th International Conference on Geomatics and Geospatial Technology (GGT 2019), 1–3 October 2019, Kuala Lumpur, Malaysia GRAVIMETRIC GEOID MODELLING OVER PENINSULAR MALAYSIA USING TWO DIFFERENT GRIDDING APPROACHES FOR COMBINING FREE AIR ANOMALY M F Pa’suya 1,2* ,A H M Din 2,3, J. C. McCubbine4 ,A.H.Omar2 , Z. M. Amin 3 and N.A.Z.Yahaya2 1Center of Studies for Surveying Science and Geomatics, Faculty of Architecture, Planning & Surveying, Universiti Teknologi MARA, Perlis, Arau Campus, 02600 Arau, Perlis - [email protected] 2 Geomatics Innovation Research Group (GNG) , 3Geoscience and Digital Earth Centre (INSTEG), Faculty of Built Environment and Surveying, Universiti Teknologi Malaysia, 81310 Johor Bahru, Johor, Malaysia- [email protected] 4 Geodesy Section, Community Safety and Earth Monitoring Division, Geoscience Australia, GPO Box 378, Canberra, ACT 2601, Australia- [email protected] KEY WORDS: LSMSA, KTH method ,gravimetric geoid, Peninsular Malaysia ABSTRACT: We investigate the use of the KTH Method to compute gravimetric geoid models of Malaysian Peninsular and the effect of two differing strategies to combine and interpolate terrestrial, marine DTU17 free air gravity anomaly data at regular grid nodes. Gravimetric geoid models were produced for both free air anomaly grids using the GOCE-only geopotential model GGM GO_CONS_GCF_2_SPW_R4 as the long wavelength reference signal and high-resolution TanDEM-X global digital terrain model. The geoid models were analyzed to assess how the different gridding strategies impact the gravimetric geoid over Malaysian Peninsular by comparing themto 172 GNSS-levelling derived geoid undulations. -
Gravity Measurements in Bolivia and Their Implications for the Tectonic Development of the Central Andean Plateau
Gravity Measurements in Bolivia and their Implications for the Tectonic Development of the Central Andean Plateau DISSERTATION Presented in Partial Fulfillment of the Requirements for the Degree Doctor of Philosophy in the Graduate School of The Ohio State University By Kevin M. Ahlgren Graduate Program in Geodetic Science The Ohio State University 2015 Dissertation Committee: Michael Bevis, Advisor Alan Saalfeld Ralph von Frese Copyright by Kevin M. Ahlgren 2015 ABSTRACT A geodetic network of gravity stations in Bolivia was established, reduced, adjusted, and analyzed. This network consists of more than 1300 relative stations with estimated precisions of less than ±0.15 mGal. The network is supported and adjusted using 15 absolute gravity stations. The motivation for observing a completely new and large gravity network in Bolivia is to contribute to the global geopotential model (GGM), which will eventually succeed EGM2008, to provide Bolivia with a much-improved height system, and to use these results to place new geophysical constraints on geodynamic models of the Central Andes. The Central Andes comprise an area of South America associated with a widening of the Andean mountain belt. The evolution of this portion of the range is not completely understood. Traditionally, models with large amounts of steady crustal shortening and thickening over a period of time from 30 Ma - 10 Ma have been hypothesized. Recently, an alternative hypothesis involving a rapid rise of the Central Andes between 10 - 6.8 Ma has been proposed. A tectonic model of this rapid rise hypothesis is developed using locally-compensated isostatic assumptions and delamination of a dense layer of eclogite in the lithospheric mantle.