South Fork Coquille Action Plan, Without Appendices
Total Page:16
File Type:pdf, Size:1020Kb
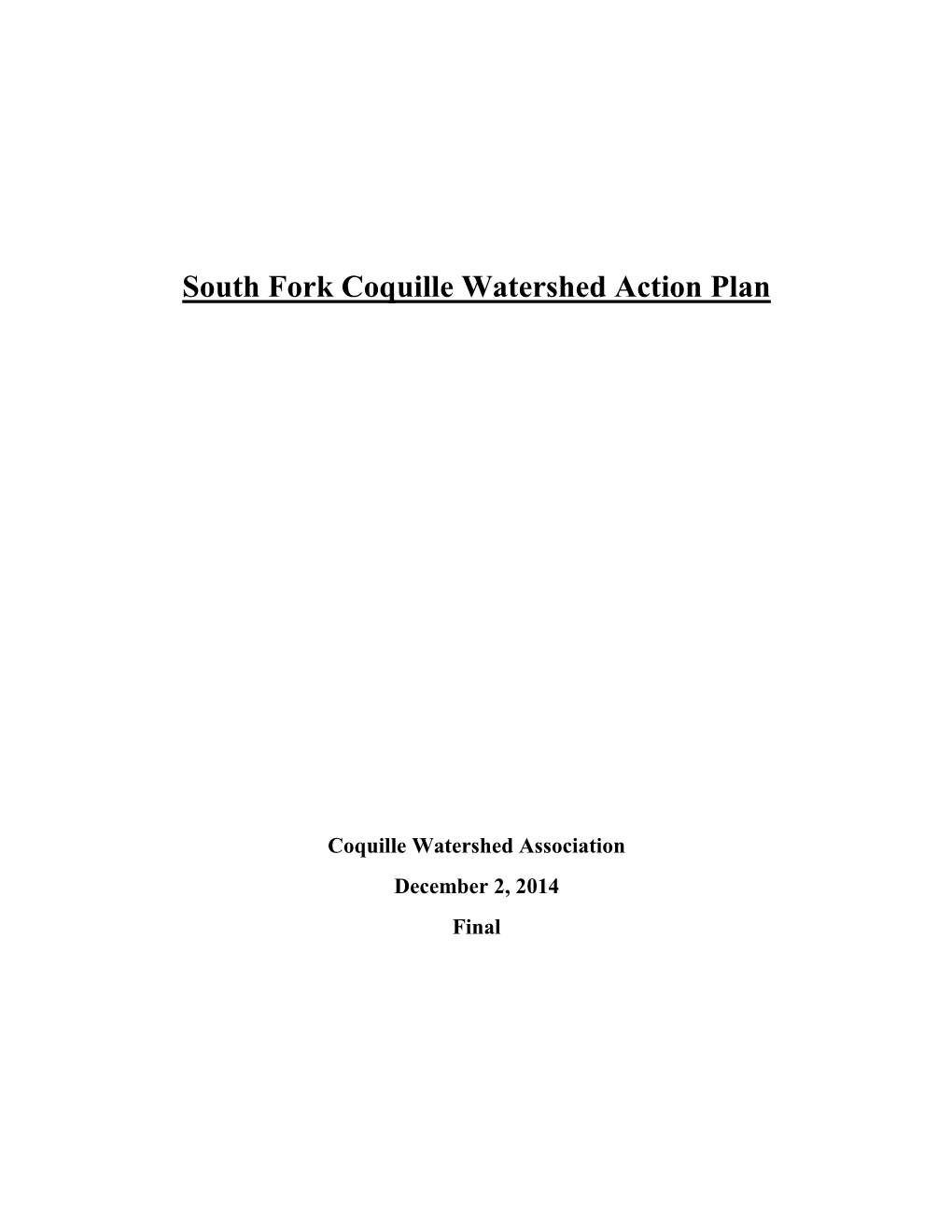
Load more
Recommended publications
-
South Fork Coquille Watershed Analysis
DOCUMENT A 13.66/2: COQUILLE fiVE, LOWER S.F. 17 10 03 00* I C 66x 1 COQUILLE RIVER, UPPER S.F 17 1:-03 01* ' United States Q, '0) Departimnt of Agriculture THIS PUBLICATION Forest Serilce CMN FE CHECKED OUT Pacific Northwest Region 1995 JA* fSouth Fork Coquille Wate1hed Analysis Iteration 1.0 Powers Ranger Distric, Slsklyou National Forest September 1995 SOUTHERN OREGON UNWVERSiTY LIBRARY ASHLAND, OREGON 97520 United Stat. Depaenent of Agnculure Forest Service Pacific Northwest Region 1995 SOUTH FORK COQUILLE WATERSHED ANALYSIS ITERATION 1.0 I have read this analysis and it meets the Standards and Guidelines for watershed analysis required by an amendment to the Forest Plan (Record of Decision dated April 1994). Any additional evidence needed to make a decision will be gathered site-specifically as part of a NEPA document or as an update to this document. SIGNED CoQ 4 DATE q 1T2 letE District Ranger Powers Ranger District Siskiyou National Forest South Fork Coquille Watershed Analysis - September 1995 Developed by Interdisciplinary Team Members: Steve Harbert Team leader Betsy Howell Wildlife Biologist Dave Shea Botantist, Wildlife Biologist Ruth Sisko Forester Cindy Ricks Geologist Chris Parks Hydrologist Max Yager Fish Biologist Kathy Helm Writer-Editor (March-April 1995), BLM Tina Harbert Writer-Editor (May-July 1995), Powers R.D. Joe Hallett Cultural Resource Key Support: Joel King Forest Planner, Siskiyou National Forest Sue Olson Acting District Ranger, Powers R.D. (Jan-May 1995) Carl Linderman District Ranger, Powers R.D. Marshall Foster GIS, Powers R.D. Jodi Shorb Computer Assistant Linda Spencer Computer Support For Further Information, contact: Powers Ranger District Powers, OR 97466 (503) 439-3011 The policy of the United States Department of Agriculture Forest Service prohibits discrimination on the basis of race, color, national origin, age, religion, sex, or disability, familial status, or political affiliation. -
Kayak & Canoe Guide to Long Island State Parks (Pdf)
KKKAYAKAYAKAYAK & C ANOEANOEANOE G GGUIDEUIDEUIDE TOTOTO L LLONGONGONG I IISLANDSLANDSLAND S SSTATETATETATE P PPARKSARKSARKS NEW YORK STATE George E. Pataki, Governor NEW YORK STATE OFFICE OF PARKS, RECREATION AND HISTORIC PRESERVATION Bernadette Castro, Commissioner LONG ISLAND STATE PARK REGION John Norbeck, Regional Director An Equal Opportunity/Affirmative Action Program Some of the best kayak and canoeing waters in New York State are Orient Beach State Park located on Long Island. Parks featuring kayak and canoe access include: (631) 323 2440 4 5 9 8 ✫ 6 7 3 1 2 Great South Bay 1. Jones Beach State Park 2. Captree State Park 3. Heckscher State Park North Shore 4. Sunken Meadow State Park 5. Nissequogue River State Park ` Lakes 6. Hempstead Lake State Park 7. Belmont Lake State Park Directions: Park located 118 miles from Manhattan at the end of Long Island’s North Fork. Take LIE (495) east to the East End 8. Hither Hills State Park (South Fork) end, then Rt. 25 east to the Park. 9. Orient Beach State Park (North Fork) Launch site access Gardiners Bay to the south and Long Saftey Tips: Beach (Hallock’s) Bay to the north. • Always wear an approved life jacket. • Use common sense. • Be aware of weather, tides and currents. Kayak drop-off is approximately halfway around the circle • Leave a float plan on your dashboard (for example: Kayaking adjacent to the parking lot. Hallock’s Bay is a 30 yard carry into Great South Bay, back around 4). and Gardiners Bay requires a 50 yard carry. • Avoid marked swimming areas. -
Community Service Plan 2014-17
Community Service Plan 2014-17 Southampton Hospital Mission Statement: The Mission of Southampton Hospital is to provide and ensure the highest quality of healthcare services for its entire community. Description of the community served: Southampton’s Service area extends from Montauk to the East to Westhampton Beach to the West and the Southern area of Riverhead in the center of the East End of Long Island. The service area accounts for approximately eight-five (85) percent of total admissions to the Hospital and includes the following zip codes: Amagansett (11930); East Hampton (11937); Bridgehampton (11932); Southampton (11968); Hampton Bays (11946); Sag Harbor (11963); Sagaponack (11962); Montauk (11954); Riverhead (11901); East Quogue (11942); Quogue (11959); Remsenburg (11960); Water Mill (11976); Westhampton Beach (11978); and Westhampton (11977). In addition, the Hospital’s service area is home to the Shinnecock Nation. Since April of 1995, Southampton Hospital has worked with the Nation to provide services at the Shinnecock Indian Health Clinic through a contract with the NYS Department of Health. On an annual basis, the Clinic provides approximately 2,000 medical and 800 dental visits for eligible members of the Shinnecock Nation. Southampton Hospital’s service area has a year-round population of approximately 115,536 residents. The population in the Hospital’s service area, a resort destination, more than doubles during the summer season when tourists and seasonal residents from all over the world come to the South Fork of Long Island. Demographics: While the South Fork has a significant second-home population, many of whom use Southampton Hospital for various services, we are addressing only the year-round population for the purpose of this plan. -
South Fork Wind Farm and South Fork Export Cable Project Biological Assessment for the National Marine Fisheries Service
South Fork Wind Farm and South Fork Export Cable Project Biological Assessment January 2021 For the National Marine Fisheries Service U.S. Department of the Interior Bureau of Ocean Energy Management Office of Renewable Energy Programs This page left blank intentionally. South Fork Wind Farm and South Fork Export Cable Project Biological Assessment for the National Marine Fisheries Service CONTENTS Introduction ................................................................................................................................................. 1 Action Area ............................................................................................................................................ 2 Renewable Energy Process ..................................................................................................................... 4 Design Envelope ..................................................................................................................................... 7 Endangered Species Act Section 7 Consultation History ....................................................................... 7 Proposed Action .......................................................................................................................................... 7 Construction ........................................................................................................................................... 8 South Fork Wind Farm ................................................................................................................... -
Motor Vehicle Use on the Rogue River-Siskiyou National Forest FSEIS
United States Department of Agriculture FINAL SUPPLEMENTAL ENVIRONMENTAL IMPACT STATEMENT Volume 1 Motorized Vehicle Use on the Rogue River-Siskiyou National Forest for the greatest good September 2015 VicinityVicinity Map Map OREGON Rogue River-Siskiyou National Forest High Cascades Powers 5 ¨¦§ Grants Pass Wild Rivers Gold Beach Medford I Siskiyou Mountains Wild Rivers OREGON CALIFORNIA The U.S. Department of Agriculture (USDA) prohibits discrimination in all its programs and activities on the basis of race, color, national origin, age, disability, and where applicable, sex, marital status, familial status, parental status, religion, sexual orientation, genetic information, political beliefs, reprisal, or because all or part of an individual's income is derived from any public assistance program. (Not all prohibited bases apply to all programs.) Persons with disabilities who require alternative means for communication of program information (Braille, large print, audiotape, etc.) should contact USDA's Lead Agency: TARGET CenterUSDA at Forest(202) 720 Service-2600 (voice and TDD). To file a complaintIn accordance of discrimination, with Federal write civil to rights USDA, law Director, and U.S. Office Department of of Civil Rights,Rogue 1400 River-Siskiyou Independence Avenue,National S.W., Forest Washington,Agriculture D.C. 20250 -(USDA)9410, or civilcall (800)rights 795 regulations-3272 (voice) and or policies, (202) the USDA, its Agencies, offices, and employees, and institutions participating in or 720-6382 (TDD). USDA is an equal -
Annual Report 2018
ANNUAL REPORT 2018 BOARD OF DIRECTORS Steve Mitchell, Chair, Hailey Lori Gibson Banducci, Vice Chair, Boise Bill Weppner, Secretary, Boise Judy Baker, Treasurer, Boise Tanya Anderson, Victor Jerry Brady, Idaho Falls Carolyn Coiner, Twin Falls Paul Cunningham, Boise Jim DeWitt, Boise David Eichberg, Boise Scott Friedman, Sun Valley Steve Lockwood, Sandpoint Jim Norton, Boise John O’Connor, Bonners Ferry Rebecca Patton, Hailey Buddy Paul, Coeur d’Alene Julie Richardson, Hailey Jerry Scheid, Idaho Falls Kim Trotter, Driggs Margrit von Braun, Moscow STAFF Natalie Chavez Communications Associate Jenny Estes Development Director ICL Artist in Residence Jessica L. Bryant. Justin Hayes Program Director Shelby Herber Community Engagement Assistant Austin Hopkins ART & NATURE Senior Conservation Associate Josh Johnson Conservation Associate ICL’s 2018 Artist in Residence Jessica L. Bryant created Rick Johnson our cover image this year as part of ICL’s celebration of Executive Director Marie Callaway Kellner the 50th anniversary of the Wild and Scenic Rivers Act. Water Associate Betsy Mizell Our artist in residence program is sponsored by ARTA Central Idaho Director River Trips and supported by an anonymous donor. The Matt Nykiel North Idaho Associate residency explores the nexus between art and nature. Mallory Parsons Accounting Assistant Jonathan Oppenheimer Jessica’s amazing and detailed landscapes have an almost- Government Relations Director To celebrate the photographic quality, and if you are lucky enough to Ben Otto Energy Associate 50th anniversary view them in person the luminosity of these watercolor John Robison of the Wild and paintings is likewise striking. Jessica committed to Public Lands Director Haley Robinson Scenic Rivers Act, supporting ICL’s work by creating a series of paintings of Development & Marketing Associate Idaho’s wild and scenic rivers. -
WAR in the WOODS! the Invasion Has Begun
Volume 34, Number 1 Spring 2015 The Southern Pine Beetle WAR IN THE WOODS! The invasion has begun. A threat Credit: Erich Vallery, US Forest Service Long Island were killed by the bigger than the worst storm, the tiny insect. Not considered an biggest fire and the most intrusive “invasive species,” because the development, looms large over the beetle is native to the United States Long Island Pine Barrens and other but has expanded its range due to regional forests. It’s the introduction global warming, the Southern Pine of the Southern Pine Beetle into Beetle has destroyed more than Long Island’s premier ecosystem. 30,000 acres of the New Jersey Forestry experts agree that Pinelands — a one million acre pine the three millimeter-long insects barrens forest. (smaller than a grain of rice) cannot Federal, state, county and town ever be eradicated and that only forestry experts are working to an unending war to control mount a defense against the their spread will prevent the tiny, but voracious insects, which loss of the native Pitch Pines can destroy a 50-foot tall pitch pine that dominate New York’s third in as little as two months. A State Forest Preserve. The primary detailed description of the beetle strategy for controlling the insects is and its capacity to kill appears to cut down affected trees and, in MINI MONSTER: The Southern Pine Beetle now threatens the Long Island Pine Barrens. in “The Thicket” on page two. some cases, those near them. Other tactics include prescribed fire and Experts agree that a management plan to try to control the infestation use of pheromones to contain and destroy the beetles. -
Natural History of Oregon Coast Mammals Chris Maser Bruce R
Forest Servile United States Depa~ment of the interior Bureau of Land Management General Technical Report PNW-133 September 1981 ser is a ~ildiife biologist, U.S. ~epa~rn e Interior, Bureau of La gement (stationed at Sciences Laboratory, Corvallis, Oregon. Science Center, ~ewpo Sciences Laborato~, Corvallis, Oregon. T. se is a soil scientist, U.S. wa t of culture, Forest Service, Pacific rthwest Forest and ange ~xperim Station, lnst~tute of orthern Forestry, Fairbanks, Alaska. Natural History of Oregon Coast Mammals Chris Maser Bruce R. Mate Jerry F. Franklin C. T. Dyrness Pacific Northwest Forest and Range Experiment Station U.S. Department of Agriculture Forest Service General Technical Report PNW-133 September 1981 Published in cooperation with the Bureau of Land Management U.S. Department of the Interior Abstract Maser, Chris, Bruce R. Mate, Jerry F. Franklin, and C. T. Dyrness. 1981. Natural history of Oregon coast mammals. USDA For. Serv. Gen. Tech. Rep. PNW-133, 496 p. Pac. Northwest For. and Range Exp. Stn., Portland, Oreg. The book presents detailed information on the biology, habitats, and life histories of the 96 species of mammals of the Oregon coast. Soils, geology, and vegetation are described and related to wildlife habitats for the 65 terrestrial and 31 marine species. The book is not simply an identification guide to the Oregon coast mammals but is a dynamic portrayal of their habits and habitats. Life histories are based on fieldwork and available literature. An extensive bibliography is included. Personal anecdotes of the authors provide entertaining reading. The book should be of use to students, educators, land-use planners, resource managers, wildlife biologists, and naturalists. -
Appendix B the Existing Transportation System Elements
Appendix B The Existing Transportation System Elements and Deficiencies B-1 THIS PAGE LEFT INTENTIONALLY BLANK B-2 THE EXISTING TRANSPORTATION SYSTEM ELEMENTS 1. Rail Transportation Existing Service and Ridership There are five train stations currently serving the Town of Southampton on the Long Island Rail Road’s Montauk Branch. These stations are located in Speonk, Westhampton, Hampton Bays, Southampton and Bridgehampton1. The train station stops at Quogue and Southampton College were discontinued in 1996 by the LIRR reportedly due to low ridership. Water Mill was previously closed. The entire Long Island Rail Road Service Map is shown in Figure B-1. Service on the Long Island Rail Road (LIRR) is summarized in Table B-1 and B-2. The additional summer service includes extra trains added primarily on Friday afternoons and evening in the eastbound direction and on Sundays and holidays in the westbound direction. Leave Penn Speonk Westhampto Hampton Southampton Bridgehampto Montauk Station n Bays n Weekday 12:35 A.M. 2:47 A.M. 2:53 A.M. 3:03 A.M. 3:13 A.M. 3:21 A.M. 3:58 A.M. 7:49 A.M. 9:44 A.M. 9:50 A.M. 10:00 A.M. 10:10 A.M. 11:18 A.M. 11:53 A.M. 11:04 A.M. 1:15 P.M. 1:21 P.M. 1:31 P.M. 1:41 P.M. 1:49 P.M. 1:59 P.M. 1:54 P.M. – -- 3:41 P.M. 3:50 P.M. 4:02 P.M. 4:10 P.M. -
Research Natural Areas in Oregon And
This file was created by scanning the printed publication. Text errors identified by the software have been corrected; however, some errors may remain. United States Department of Research Natural Areas in Agriculture Forest Service Oregon and Washington: Pacific Northwest Research Station Past and Current Research General Technical Report PNW-197 and Related Literature November 1986 Sarah E. Greene, Tawny Blinn, and Jerry F. Franklin I Authors SARAH E. GREENE is a research forester. TAWNY BLINN is an editorial assistant. and JERRY F. FRANKLIN is a chief plant ecologist. U.S. Department of Agriculture. Forest Service. Pacific Northwest Research Station. Forestry Sciences Laboratory. 3200 Jefferson Way. Corvallis, Oregon 97331. Foreword In 1971, I joined the Pacific Northwest Forest and Range Exper- iment Station as Station Director and, among other duties, be- came chainman of the Interagency Committee on Research Natural Areas. It was a chair that I held for 4 years, and it is a - - pleasure to reflect, more than 10 years later, on the progress that has been made. Oregon and Washington already had a vigorous program of preser- vation of Natural Areas for scientific and educational purposes in 1971. In preparation at that time were several publications important to identifying and protecting Natural Areas, including a description of natural vegetation of Oregon and Washington (Franklin and Dyrness 1973), an inventory of Federal Research Natural Areas in Oregon and Washington (Franklin and others 1972),1/ and a comprehensive inventory of Natural Areas rec- ognized by the Society of American Foresters (Buckman and Quintus 1972). The Interagency Committee, with participation from The Nature Conservancy and the States of Oregon and Washington then asked, "What should a well-balanced program of Research Natural Area preservation include?" This led to the publication, "Research Natural Area Needs in the Pacific Northwest: A Contribution to Land-Use Planning" (Dyrness and others 1975). -
South Fork Kent Creek Watershed
OLSON ECOLOGICAL SOLUTIONS South Fork Kent Creek Watershed Watershed Plan 8/1/2020 This report was prepared using United States Environmental Protection Agency funds under Section 319 of the Clean Water Act distributed through the Illinois Environmental Protection Agency. The findings and recommendations contained herein are not necessarily those of the funding agencies. Olson Ecological Solutions: 2221 Hammond Drive, Schaumburg, IL 60173 t) 815.985.2689 [email protected] South Fork Kent Creek Watershed Plan November 2020 Section 2 Acknowledgements The South Fork Kent Creek Watershed Plan was initiated by efforts of the Rockford Park District (RPD). RPD has been working to maintain and improve the recreational water quality of Levings Lake for many years. In 2016, they enlisted Olson Ecological Solutions (OES), and JadEco Natural Resource Consultation and Management to create a report, entitled A Cleaner Levings Lake: Putting Nature to Work, identifying water quality issues and solutions to improve Levings Lake water quality (OES, 2017). RPD has implemented the following recommendations from this report: 20 BioHaven® floating islands, vegetated filter strips, a 0.64-acre constructed stormwater wetland, and native plantings. A scope limitation acknowledged in this report is that projects for water quality improvement could only be made on Rockford Park District owned property. For a more sustainable and comprehensive solution, RPD needed to consider the entire watershed, especially areas upstream of the lake. With this in mind, RPD decided to move forward with developing a more comprehensive watershed wide plan. RPD applied for and received funding from the Illinois Environmental Protection Agency through the Section 319 Nonpoint Source Pollution Control Financial Assistance Program. -
Scoping Summary Report for the South Fork Wind Farm Environmental Impact Statement
Scoping Summary Report for the South Fork Wind Farm Environmental Impact Statement JANUARY 2021 PREPARED FOR Bureau of Ocean Energy Management PREPARED BY SWCA Environmental Consultants Scoping Summary Report for the South Fork Wind Farm Environmental Impact Statement CONTENTS 1 Introduction .......................................................................................................................................... 1 2 Objective ................................................................................................................................................ 1 3 Methodology .......................................................................................................................................... 1 3.1 Terminology ................................................................................................................................. 1 3.2 Comment Submittal ...................................................................................................................... 2 3.3 Comment Processing .................................................................................................................... 2 Compilation of Submissions .................................................................................................... 2 Identification of Comments ..................................................................................................... 3 4 Scoping Submission and Comment Summary ..................................................................................