A Novel Flight Style Allowing the Smallest Featherwing Beetles to Excel
Total Page:16
File Type:pdf, Size:1020Kb
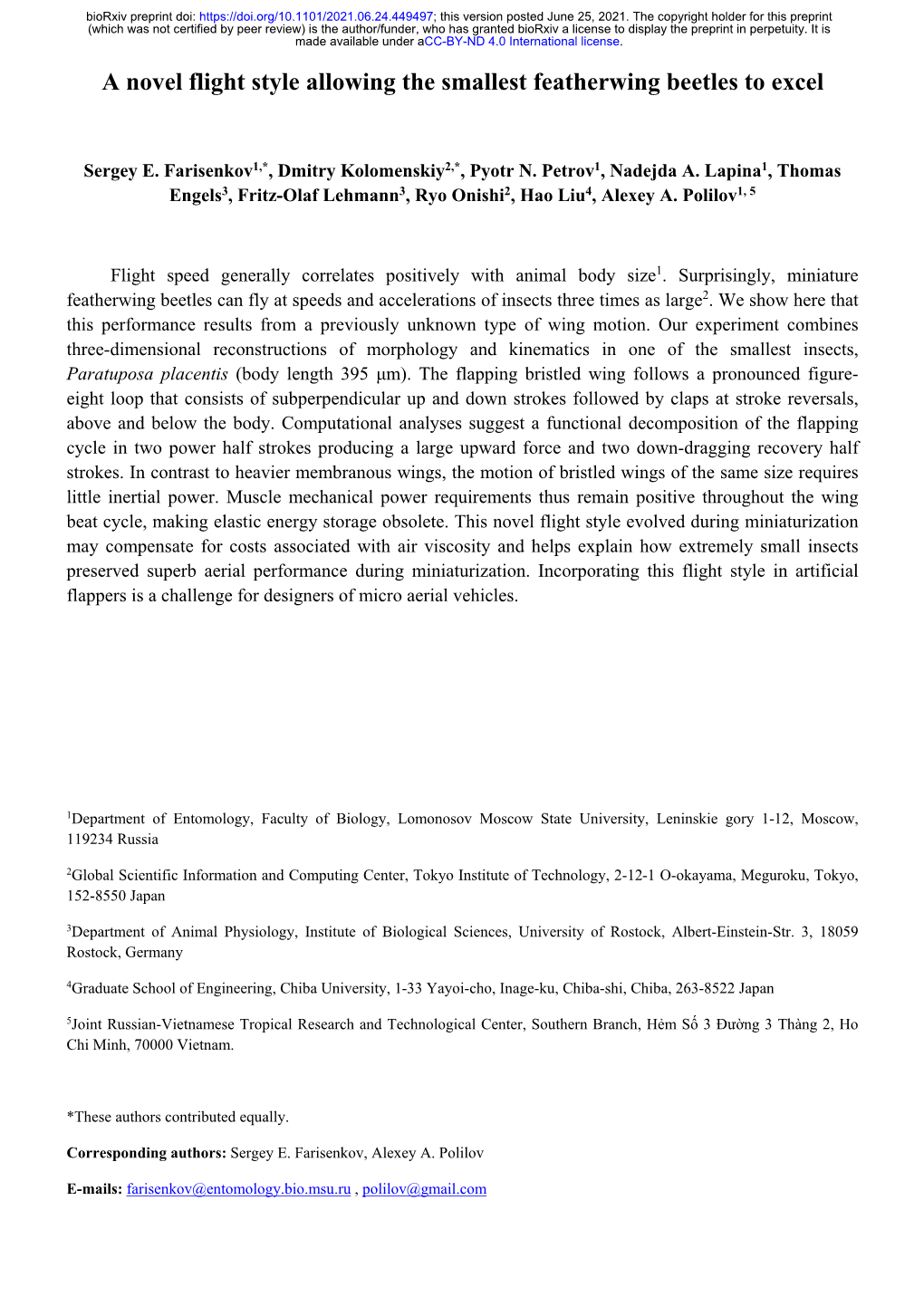
Load more
Recommended publications
-
The Phylogeny of Ptiliidae (Coleoptera: Staphylinoidea) – the Smallest Beetles and Their Evolutionary Transformations
77 (3): 433 – 455 2019 © Senckenberg Gesellschaft für Naturforschung, 2019. The phylogeny of Ptiliidae (Coleoptera: Staphylinoidea) – the smallest beetles and their evolutionary transformations ,1, 2 3 4 Alexey A. Polilov* , Ignacio Ribera , Margarita I. Yavorskaya , Anabela Cardoso 3, Vasily V. Grebennikov 5 & Rolf G. Beutel 4 1 Department of Entomology, Biological Faculty, Lomonosov Moscow State University, Moscow, Russia; Alexey A. Polilov * [polilov@gmail. com] — 2 Joint Russian-Vietnamese Tropical Research and Technological Center, Hanoi, Vietnam — 3 Institute of Evolutionary Biology (CSIC-Universitat Pompeu Fabra), Barcelona, Spain; Ignacio Ribera [[email protected]]; Anabela Cardoso [[email protected]] — 4 Institut für Zoologie und Evolutionsforschung, FSU Jena, Jena, Germany; Margarita I. Yavorskaya [[email protected]]; Rolf G. Beutel [[email protected]] — 5 Canadian Food Inspection Agency, Ottawa, Canada; Vasily V. Grebennikov [[email protected]] — * Cor- responding author Accepted on November 13, 2019. Published online at www.senckenberg.de/arthropod-systematics on December 06, 2019. Published in print on December 20, 2019. Editors in charge: Martin Fikáček & Klaus-Dieter Klass. Abstract. The smallest beetles and the smallest non-parasitic insects belong to the staphylinoid family Ptiliidae. Their adult body length can be as small as 0.325 mm and is generally smaller than 1 mm. Here we address the phylogenetic relationships within the family using formal analyses of adult morphological characters and molecular data, and also a combination of both for the frst time. Strongly supported clades are Ptiliidae + Hydraenidae, Ptiliidae, Ptiliidae excl. Nossidium, Motschulskium and Sindosium, Nanosellini, and a clade comprising Acrotrichis, Smicrus, Nephanes and Baeocrara. A group comprising Actidium, Oligella and Micridium + Ptilium is also likely monophy- letic. -
Green-Tree Retention and Controlled Burning in Restoration and Conservation of Beetle Diversity in Boreal Forests
Dissertationes Forestales 21 Green-tree retention and controlled burning in restoration and conservation of beetle diversity in boreal forests Esko Hyvärinen Faculty of Forestry University of Joensuu Academic dissertation To be presented, with the permission of the Faculty of Forestry of the University of Joensuu, for public criticism in auditorium C2 of the University of Joensuu, Yliopistonkatu 4, Joensuu, on 9th June 2006, at 12 o’clock noon. 2 Title: Green-tree retention and controlled burning in restoration and conservation of beetle diversity in boreal forests Author: Esko Hyvärinen Dissertationes Forestales 21 Supervisors: Prof. Jari Kouki, Faculty of Forestry, University of Joensuu, Finland Docent Petri Martikainen, Faculty of Forestry, University of Joensuu, Finland Pre-examiners: Docent Jyrki Muona, Finnish Museum of Natural History, Zoological Museum, University of Helsinki, Helsinki, Finland Docent Tomas Roslin, Department of Biological and Environmental Sciences, Division of Population Biology, University of Helsinki, Helsinki, Finland Opponent: Prof. Bengt Gunnar Jonsson, Department of Natural Sciences, Mid Sweden University, Sundsvall, Sweden ISSN 1795-7389 ISBN-13: 978-951-651-130-9 (PDF) ISBN-10: 951-651-130-9 (PDF) Paper copy printed: Joensuun yliopistopaino, 2006 Publishers: The Finnish Society of Forest Science Finnish Forest Research Institute Faculty of Agriculture and Forestry of the University of Helsinki Faculty of Forestry of the University of Joensuu Editorial Office: The Finnish Society of Forest Science Unioninkatu 40A, 00170 Helsinki, Finland http://www.metla.fi/dissertationes 3 Hyvärinen, Esko 2006. Green-tree retention and controlled burning in restoration and conservation of beetle diversity in boreal forests. University of Joensuu, Faculty of Forestry. ABSTRACT The main aim of this thesis was to demonstrate the effects of green-tree retention and controlled burning on beetles (Coleoptera) in order to provide information applicable to the restoration and conservation of beetle species diversity in boreal forests. -
31 First Record of Batocera Rufomaculata (De Geer, 1775) from Sunderban Biosphere Reserve, West Bengal
International Journal of Entomology Research ISSN: 2455-4758 www.entomologyjournals.com Volume 1; Issue 3; March 2016; Page No. 31-32 First record of Batocera rufomaculata (De Geer, 1775) from Sunderban biosphere reserve, West Bengal 1 Bulganin Mitra, 2 Udipta Chakraborti, 3 Olive Biswas, 4 Sankarsan Roy, 5 Kaushik Mallick, 6 Priyanka Das 1, 2, 3, 4, 6 Zoological Survey of India, Prani Vigyan Bhawan, M-Block, New Alipore, Kolkata. 5 Post Graduate Department of Zoology, Asutosh College, Kolkata Abstract Studies on Longhorn beetles (Coleoptera) in Sunderban region is very poor. Altogether, 8 species under 3 subfamilies are already reported from Sunderban Biosphere Reserve. Present communication reports Batocera rufomaculata (De Geer, 1775) for the first time from this Biosphere reserve. Keywords: Sunderban Biosphere Reserve, Cerambycidae, Lamiinae, Batocera Introduction Sunderban region in India is 9600 sq km (4200 sq km of Reserved Forest and 5400 sq km of non-forest, inhabited region) which constitutes the Sunderban Biosphere Reserve (SBR). Indian Sunderban is bound on the west by river Muriganga and on the east by rivers Harinbhahga and Raimangal. Administrative boundary of the Sunderban is spread over two districts i.e. North 24-Parganas (Hingalganj, Hasnabad, Haroa, Sandeskhali - I,II, and Minakhan blocks) and South 24-Parganas (Sagar, Namkhana, Kakdwip, Patharpratima, Kultali, Mathurapur-I,II, Jaynagar-I,II, Canning-I,II, Basanti and Gosaba blocks).The extent of mangrove Reserve Forests in Indian Sunderban is around 4260 sq km, out of which 55% is under land vegetation cover and balance 45% is under water body/ inter-tidal zone. Studies on beetles and weevils (Coleoptera) in Sunderban region is very poor. -
(Coleoptera) in the Babia Góra National Park
Wiadomości Entomologiczne 38 (4) 212–231 Poznań 2019 New findings of rare and interesting beetles (Coleoptera) in the Babia Góra National Park Nowe stwierdzenia rzadkich i interesujących chrząszczy (Coleoptera) w Babiogórskim Parku Narodowym 1 2 3 4 Stanisław SZAFRANIEC , Piotr CHACHUŁA , Andrzej MELKE , Rafał RUTA , 5 Henryk SZOŁTYS 1 Babia Góra National Park, 34-222 Zawoja 1403, Poland; e-mail: [email protected] 2 Pieniny National Park, Jagiellońska 107B, 34-450 Krościenko n/Dunajcem, Poland; e-mail: [email protected] 3 św. Stanisława 11/5, 62-800 Kalisz, Poland; e-mail: [email protected] 4 Department of Biodiversity and Evolutionary Taxonomy, University of Wrocław, Przybyszewskiego 65, 51-148 Wrocław, Poland; e-mail: [email protected] 5 Park 9, 42-690 Brynek, Poland; e-mail: [email protected] ABSTRACT: A survey of beetles associated with macromycetes was conducted in 2018- 2019 in the Babia Góra National Park (S Poland). Almost 300 species were collected on fungi and in flight interception traps. Among them, 18 species were recorded from the Western Beskid Mts. for the first time, 41 were new records for the Babia Góra NP, and 16 were from various categories on the Polish Red List of Animals. The first certain record of Bolitochara tecta ASSING, 2014 in Poland is reported. KEY WORDS: beetles, macromycetes, ecology, trophic interactions, Polish Carpathians, UNESCO Biosphere Reserve Introduction Beetles of the Babia Góra massif have been studied for over 150 years. The first study of the Coleoptera of Babia Góra was by ROTTENBERG th (1868), which included data on 102 species. During the 19 century, INTERESTING BEETLES (COLEOPTERA) IN THE BABIA GÓRA NP 213 several other papers including data on beetles from Babia Góra were published: 37 species were recorded from the area by KIESENWETTER (1869), a single species by NOWICKI (1870) and 47 by KOTULA (1873). -
The Tropical Fig Borer, Batocera Rufomaculata (Coleoptera: Cerambycidae), New for Turkey
The Tropical Fig Borer, Batocera rufomaculata (Coleoptera: Cerambycidae), new for Turkey by Göksel Tozlu and Hikmet Özbek Abstract: The Tropical Fig Borer, Batocera rufomaculata (De Geer, 1775) is recorded from the eastern Mediterranean Region of Turkey as a genus, species and a fig pest new for Turkey. The material collected and the views of some growers suggest that B. rufomaculata was probably in- troduced to Turkey from Israel, Lebanon, Syria or Iraq in the 1970s. Kurzfassung: Der Tropische Feigenbohrer, Batocera rufomaculata (De Geer, 1775) wird erst- mals aus der Türkei gemeldet, und zwar aus der östlichen Mittelmeeregion. Nicht nur die Art, auch der Genus ist neu für die Türkei; auch als Feigenschädling wird die Art in der Türkei erst- mals registriert. Aufgrund des gesammelten Materials und aufgrund von Berichten von Feigenan- bauern wird geschlossen, daß B. rufomaculata in die Türkei wahrscheinlich aus Israel, dem Liba- non, Syrien oder dem Irak eingeschleppt wurde. Key words: Batocera rufomaculata, Cerambycidae, fig borer, new record, new pest, alien spe- cies, Turkey, Middle East. Introduction The Tropical Fig Borer, Batocera rufomaculata (De Geer, 1775), has a tropical distribution, extending from southern China through Malaya, India, Sri Lanka, Madagascar and Mauritius to eastern Africa (AVIDOV & HARPAZ 1969, KATBEH-BADER 1996). This pest species was introduced into Israel in 1949 (AVIDOV & HARPAZ 1969) and into Jordan in the 1940s (KAT- BEH-BADER 1996). Following this, HEYROVSKY (1963) recorded the presence of this species in Jordan in 1957. Although HALPERIN & HOLZSCHUH (1993) indicated that B. rufomaculata has been disappearing in Israel since the 1970s, more recently KATBEH-BADER (pers. -
(Coleoptera: Ptiliidae): Description of New Taxa and Phylogenetic Analysis
Systematic Entomology Systematic Entomology (2009), 34, 113–136 Discheramocephalini, a new pantropical tribe of featherwing beetles (Coleoptera: Ptiliidae): description of new taxa and phylogenetic analysis VASILY V. GREBENNIKOV1,2 1Entomology Research Laboratory, Ottawa Plant Laboratories, Canadian Food Inspection Agency, Ottawa, Ontario, Canada and 2Entomology Group, Institut fu¨ r Spezielle Zoologie und Evolutionbiologie, Friedrich-Schiller-Universita¨ t, Jena, Germany Abstract. Two new genera and eight new species of featherwing beetles (Cole- optera: Ptiliidae) possessing a remarkable horizontal perforation of the mesoven- tral keel are described: Skidmorella vietnamensis sp.n. (Vietnam), S. memorabilis sp.n. (Indonesia), S. serrata sp.n. (Vietnam), Fenestellidium capensis gen. et sp.n. (South Africa, type species), F. kakamegaensis sp.n. (Kenya), Cissidium okuensis sp.n. (Cameroon), Dacrysoma usambarensis gen. et sp.n. (Tanzania, type species) and D. felis sp.n. (Madagascar). A phylogenetic analysis of 24 taxa and 37 parsimony-informative characters supports the hypothesis of a single origin of the mesoventral perforation, thus uniting Discheramocephalus, Skidmorella, Africop- tilium, Fenestellidium, Cissidium and Dacrysoma into a pantropically distributed clade, for which a new tribe Discheramocephalini (type genus Discheramocephalus) is proposed. Identification keys to Discheramocephalini genera and, in some cases, to species are provided. Each new species is illustrated with scanning electron microscopy images. Introduction of such groups. This implies that among small insects there are many new taxa to be discovered and described. The family Ptiliidae comprises beetles with the smallest body This assumption of taxonomic obscurity was correct for length and volume: the length of most species is between 0.6 the Ptiliidae I collected recently in South Africa, Kenya, and 1.2 mm, with a reported minimum of about 0.40 mm Tanzania and Cameroon. -
Management of Insect Pests and Diseases of Jackfruit (Artocarpus
Acta Entomology and Zoology 2021; 2(1): 37-46 E-ISSN: 2708-0021 P-ISSN: 2708-0013 www.actajournal.com Management of insect pests and diseases of jackfruit AEZ 2021; 2(1): 37-46 Received: 19-11-2020 (Artocarpus heterophyllus L.) in agroforestry system: Accepted: 24-12-2020 A review Ahasan Ullah Khan (1) Department of Entomology, Faculty of Agriculture, Sylhet Agricultural University, Sylhet, Ahasan Ullah Khan, Md. Abdur Razzak Choudhury, Md. Abdul Bangladesh Maleque, Chandra Kanta Dash, Mohammad Samiul Ahsan Talucder, (2) Climate-Smart Agriculture Lab, Department of Agroforestry and Abu Rashed Md. Maukeeb, Israt Jahan Ema and Muhammad Adnan Environmental Science, Faculty of Agriculture, Sylhet Agricultural University, Sylhet-3100, Bangladesh DOI: https://doi.org/10.33545/27080013.2021.v2.i1a.29 Md. Abdur Razzak Choudhury Department of Entomology, Faculty Abstract of Agriculture, Sylhet Agricultural The main aim of this review is to document the insect pests and diseases of jackfruit (Artocarpus University, Sylhet, Bangladesh heterophyllus L.) and their management in Bangladesh compared to other jackfruit growing countries. Md. Abdul Maleque This article was based on mostly literature review. A. heterophyllus being the national fruit of Department of Entomology, Faculty Bangladesh, is widely consumed by most of the rural people. All parts of the fruit and tree are used as of Agriculture, Sylhet Agricultural human food, animal feed and wood source for furniture. Jackfruit contains anti-bacterial, anti-diabetic, University, Sylhet, Bangladesh anti-oxidant, anti-inflammatory and anti-helminthic properties. The fruit is rich in carbohydrates, Chandra Kanta Dash minerals, carboxylic acids, dietary fiber, vitamins and minerals. The seed is rich in manganese, Department of Entomology, Faculty magnesium, potassium, calcium iron and lectins and thus meets up nutritional requirements for the of Agriculture, Sylhet Agricultural University, Sylhet, Bangladesh rural people. -
Изучение Ассоциативного Обучения Nephanes Titan (Newman, 1834) И Других Перокрылок (Coleoptera: Ptiliidae)
Московский Государственный Университет им . М.В. Ломоносова Биологический факультет Кафедра энтомологии Московская гимназия на Юго -Западе № 1543 Изучение ассоциативного обучения Nephanes titan (Newman, 1834) и других перокрылок (Coleoptera: Ptiliidae) Работу выполнила : Ульяна Колесникова Научный руководитель : д.б.н. Алексей Алексеевич Полилов Москва , 2017 Введение Миниатюризация – одно из направлений в эволюции животных (Hanken, Wake, 1993) и один из основных трендов эволюции насекомых , в результате которого некоторые насекомые по размерам становятся сопоставимы с одноклеточными организмами (Четвериков , 1915). Маленькие размеры тела сильно влияют на морфологию и физиологию насекомого (Schmidt-Nielsen, 1987). С уменьшением размеров тела изменениям подвергаются все системы тела , в том числе нервная система : происходит олигомеризация и концентрация ганглиев , но относительный объем ЦНС при этом повышается . У человека относительный объем мозга – 2%, у колибри – 8%; у пчелы Apis sp . – 0,57%, у Nephanes titan – 5%, у личинки Liposcelis sp. (книжного сеноеда ) самый высокий церебральный индекс – 12%(Polilov, 2015). Семейство Ptiliidae Heer, 1843 (перокрылки ) включает в себя мельчайших жуков и мельчайших свободно живущих насекомых . Длина тела Scydosella musawasensis Hall, 1999, мельчайшего известного на сегодня жесткокрылого , составляет всего 300 мкм (Polilov, 2015). У перокрылок сокращается число обонятельных рецепторов и фасеток и сильно уменьшается относительный объем структур , отвечающих за обработку сигналов от зрительных и обонятельных рецепторов . Для имаго характерно наличие асимметричных дистальных выростов протоцеребрума ( левый больше правого ). Изменению подвергаются чашечки грибовидных тел ( Макарова , 2013), которые у насекомых отвечают , в том числе , за обучение . Грибовидные тела очень хорошо развиты у перепончатокрылых и таракановых ; у общественных насекомых степень развития грибовидных тел может варьировать внутри вида ( у рабочих пчел они гораздо больше , чем у маток или трутней ) (Strausfield et al., 1998). -
Coleoptera: Cerambycidae) of Assam, India
Rec. zool. Surv. India: Vol. 117(1)/ 78-90, 2017 ISSN (Online) : (Applied for) DOI: 10.26515/rzsi/v117/i1/2017/117286 ISSN (Print) : 0375-1511 An updated list of cerambycid beetles (Coleoptera: Cerambycidae) of Assam, India Bulganin Mitra1*, Udipta Chakraborti1, Kaushik Mallick1, Subhrajit Bhaumik2 and Priyanka Das1 1Zoological Survey of India, Prani Vigyan Bhavan, M-Block, New Alipore, Kolkata – 700 053, West Bengal, India; [email protected] 2Post Graduate, Department of Zoology, Vidyasagar College, Kolkata – 700006, West Bengal, India Abstract consolidated updated list of cerambycid fauna of Assam and reports 95 species, 64 genera, 32 tribes and 3 subfamilies. AmongAssam isthe a threestate subfamiliesin North-East from India Assam, which subfamily is considered Lamiinae as shares a biological 49 species, hotspot. followed Present by the communication subfamily Cerambycinae is the first with 38 species and Prioninae with only 8 species. Keywords: Longhorn beetle, Assam, North-East India Introduction world, therefore this beetle family is considered as one of important coleopteran family (Agarwala & Bhattacharjee, The study on long horned beetles from the northeast 2012). This communication is the first updated Indian state Assam is very poor with many species consolidated list of cerambycid beetles from the state of awaiting discovery, study and description. Among the Assam (after complete separation from other states of NE seven sister states, cerambycid fauna of Arunachal India in 1987) which includes 95 species under 64 genera Pradesh, Tripura, Meghalaya, Manipur, Mizoram, of 32 tribes belonging to 3 subfamilies along with their Nagaland are mostly worked out by the Zoological Survey distribution. of India and some other universities and institutions. -
WRA.Datasheet.Template
Assessment date 16 October 2018 Prepared by Young and Lieurance Ficus carica ALL ZONES Answer Score 1.01 Is the species highly domesticated? n 0 1.02 Has the species become naturalised where grown? 1.03 Does the species have weedy races? 2.01 Species suited to Florida's USDA climate zones (0-low; 1-intermediate; 2-high) 2 North Zone: suited to Zones 8, 9 Central Zone: suited to Zones 9, 10 South Zone: suited to Zone 10 2.02 Quality of climate match data (0-low; 1-intermediate; 2-high) 2 2.03 Broad climate suitability (environmental versatil+B8:B24ity) y 1 2.04 Native or naturalized in habitats with periodic inundation y North Zone: mean annual precipitation 50-70 inches Central Zone: mean annual precipitation 40-60 inches South Zone: mean annual precipitation 40-60 inches 1 2.05 Does the species have a history of repeated introductions outside its natural range? y 3.01 Naturalized beyond native range y 2 3.02 Garden/amenity/disturbance weed unk 3.03 Weed of agriculture n 0 3.04 Environmental weed y 4 3.05 Congeneric weed y 2 4.01 Produces spines, thorns or burrs n 0 4.02 Allelopathic n 0 4.03 Parasitic n 0 4.04 Unpalatable to grazing animals n -1 4.05 Toxic to animals n 0 4.06 Host for recognised pests and pathogens y 1 4.07 Causes allergies or is otherwise toxic to humans y 1 4.08 Creates a fire hazard in natural ecosystems unk 0 4.09 Is a shade tolerant plant at some stage of its life cycle n 0 4.10 Grows on infertile soils (oligotrophic, limerock, or excessively draining soils). -
Relative Susceptibility of Cashew Stem and Root Borers (CSRB), Plocaederus Spp
Journal of Biological Control , 26 (1): 23-28, 2012 Research Article Relative susceptibility of cashew stem and root borers (CSRB), Plocaederus spp. and Batocera rufomaculata (De Geer) (Coleoptera: Cerambycidae) to entomopathogenic nematodes P. VASANTHI. * and T. N. RAVIPRASAD Directorate of Cashew Research, Puttur 574202, Karnataka, India Corresponding author E-mail : vasan [email protected] ABSTRACT : Cashew stem and root borers (CSRB) viz., Plocaederus ferrugenius L., Plocaederus obesus Gahan and Batocera rufomaculata De Geer are major pests of cashew ( Anacardium occidentale L.) in all cashew growing tracts of India. The grubs of these CSRB species damage the vascular tissues by internal tunneling, thereby gradually killing the infested trees leading to decline in tree density. Studies were conducted at Directorate of Cashew Research, Puttur during 2010-11 to evaluate the effectiveness of entomopathogenic nematodes (EPN), Heterorhabditis indica Poinar (Rhabditida : Heterorhabditidae), Steinernema abbasi Elawad (Rhabditida : Steinernematidae) and Steinernema bicornutum Tallosi (Rhabditida : Steinernematidae) against the grubs of Plocaederus spp. and B. rufomaculata . All the three species of EPN induced mortality of Plocaederus spp. grubs in a mean duration of 14.11, 12.88 1*+$8PHj_$+12(?$'#()#%!,A#52H$$@"#$2&/*-#'$-'/4($7ok:$+12(< of Plocaederus spp. showed equal susceptibility to all the three speices of EPN . In case of grubs of B. rufomaculata, H. indica induced mortality within a mean duration of 7.43 days, which was superior than mortality induced by S. abbasi (18.25 days) and by S. bicornutum (17.94 days). It was noticed that the body weight was strongly correlated to emergence of IJs in all the three spp. of EPN. -
60 Bionomics of Batocera Rufomaculata De Geer
Bionomics of Batocera rufomaculata De Geer (Coleoptera: Cerambycidae) in mulberry farms of Jammu and Kashmir (India) Altaf Hussain Mir Post Graduate Department of Zoology, University of Kashmir, Srinagar-190006 [email protected] Abstract: Investigations on the bionomics of Batocera rufomaculata De Geer (Coleoptera: Cerambycidae) in mulberry farms of Jammu and Kashmir state (India) demonstrated an annual life cycle with an adult emergence period of over four months from May to September; adult emergence started in May, peaked in June and ended in August. Adults required maturation feeding period of 14.00±0.561 days. Sexually mature beetles mated promiscuously and copulation period averaged 60.50±6.23 seconds. Females deposited eggs singly into the bark of host plants with an average daily and life time fecundity of 1.27 and 148.66 eggs respectively. Eggs hatched in 10.20± 1.25 days and larvae developed through 9 instars in 253.35±4.37 days. Ultimate larval instar pupated in the pupal cells, made by the ultimate larvae; adults emerged from the pupal cells after 27.5±1.35 days through circular emergence holes. Larvae as well as adults caused damage to the host plants; the latter compensated the damage caused by adults to some extent by sprouting lateral buds while as grubs caused irreparable damage and lead the host plants to death. The extent of damage caused to the host plants is also reported in the paper. [Altaf Hussain Mir. Bionomics of Batocera rufomaculata De Geer (Coleoptera: Cerambycidae) in mulberry farms of Jammu and Kashmir (India).Life Sci J 2021;18 (5):60-72].